Nutrient Removal and Fouling Reduction in Electrokinetic Membrane Bioreactor at Various Temperatures
Total Page:16
File Type:pdf, Size:1020Kb
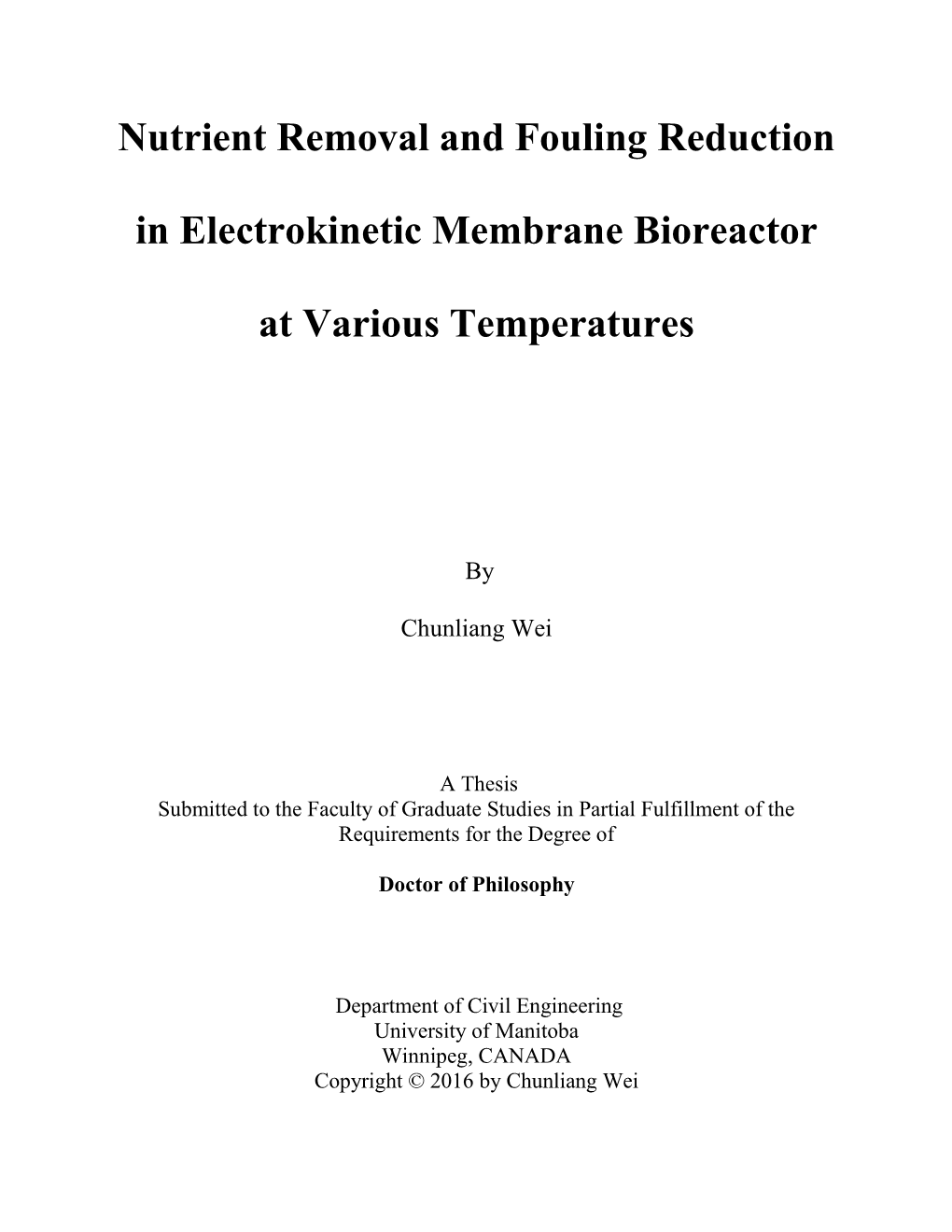
Load more
Recommended publications
-
Ultracentrifugation Techniques for the Ordering of Nanoparticles
nanomaterials Review Ultracentrifugation Techniques for the Ordering of Nanoparticles Xufeng Xu 1,† and Helmut Cölfen 2,* 1 Laboratory of Physical Chemistry, Department of Chemical Engineering and Chemistry, Eindhoven University of Technology, 5612AE Eindhoven, The Netherlands; [email protected] 2 Physical Chemistry, University of Konstanz, Universitätsstraße 10, Box 714, 78457 Konstanz, Germany * Correspondence: [email protected] † Present address: Institute of Materials, École Polytechnique Fédérale de Lausanne, 1015 Lausanne, Switzerland. Abstract: A centrifugal field can provide an external force for the ordering of nanoparticles. Especially with the knowledge from in-situ characterization by analytical (ultra)centrifugation, nanoparticle ordering can be rationally realized in preparative (ultra)centrifugation. This review summarizes the work back to the 1990s, where intuitive use of centrifugation was achieved for the fabrication of colloidal crystals to the very recent work where analytical (ultra)centrifugation is employed to tailor-make concentration gradients for advanced materials. This review is divided into three main parts. In the introduction part, the history of ordering microbeads in gravity is discussed and with the size of particles reduced to nanometers, a centrifugal field is necessary. In the next part, the research on the ordering of nanoparticles in analytical and preparative centrifugation in recent decades is described. In the last part, the applications of the functional materials, fabricated from centrifugation-induced nanoparticle superstructures are briefly discussed. Keywords: centrifugation; sedimentation; nanoparticle; concentration gradient; non-equilibrium process; superstructure; functional material Citation: Xu, X.; Cölfen, H. Ultracentrifugation Techniques for 1. Introduction the Ordering of Nanoparticles. 1.1. The Era of Microbeads in Gravity Nanomaterials 2021, 11, 333. -
Investigation of Fouling Mechanisms on Ion Exchange Membranes During Electrolytic Separations
INVESTIGATION OF FOULING MECHANISMS ON ION EXCHANGE MEMBRANES DURING ELECTROLYTIC SEPARATIONS By Matthew James Edwards A thesis submitted to the faculty of The University of Mississippi in partial fulfillment of the requirements of the Sally McDonnell Barksdale Honors College. Oxford, MS 2019 Approved by: __________________________________ Advisor: Dr. Alexander M. Lopez _________________________________ Reader: Dr. Adam Smith __________________________________ Reader: Dr. John H. O’Haver i Ó 2019 Matthew James Edwards ALL RIGHTS RESERVED ii DEDICATION I would like to dedicate this Capstone Project to my parents, Michael and Nidia Edwards. Their support and commitment to my education has been unfailing for as long as I can remember. I am thankful for everything they have done. It is with their help that I am privileged to attend The University of Mississippi, and I will forever be grateful. iii ACKNOWLEDGEMENTS I would first like thank Dr. Alexander M. Lopez and The University of Mississippi Chemical Engineering Department for the opportunity to work on this research project. The guidance, patience, and willingness to work with and teach an undergraduate student has been beneficial and inspiring to me during my time here at Ole Miss. Second, I would like to thank Dr. Paul Scovazzo for providing guidance on how to write this thesis and for allowing me to use his lab and equipment as well. I would also like to thank all the graduate students of the Chemical Engineering Department, primarily Saloumeh Kolahchyan. The willingness to take the time to answer my questions, to guide me in how use all the equipment in the lab, and to show me how to follow lab protocols required for the completion of my thesis research. -
Fractionation of Proteins with Two-Sided Electro-Ultrafiltration
Journal of Biotechnology 128 (2007) 895–907 Fractionation of proteins with two-sided electro-ultrafiltration Tobias Kappler¨ ∗, Clemens Posten University of Karlsruhe, Institute of Engineering in Life Sciences, Division Bioprocess Engineering, Kaiserstr. 12, Geb. 30.70, 76128 Karlsruhe, Germany Received 7 June 2006; received in revised form 22 December 2006; accepted 2 January 2007 Abstract Downstream processing is a major challenge in bioprocess industry due to the high complexity of bio-suspensions itself, the low concentration of the product and the stress sensitivity of the valuable target molecules. A multitude of unit operations have to be joined together to achieve an acceptable purity and concentration of the product. Since each of the unit operations leads to a certain product loss, one important aim in downstream-research is the combination of different separation principles into one unit operation. In the current work a dead-end membrane process is combined with an electrophoresis operation. In the past this concept has proven successfully for the concentration of biopolymers. The present work shows that using different ultrafiltration membranes in a two-sided electro-filter apparatus with flushed electrodes brought significant enhancement of the protein fractionation process. Due to electrophoretic effects, the filtration velocity could be kept on a very high level for a long time, furthermore, the selectivity of a binary separation process carried out exemplarily for bovine serum albumin (BSA) and lysozyme (LZ) could be greatly increased; in the current case up to a value of more than 800. Thus the new two-sided electro-ultrafiltration technique achieves both high product purity and short separation times. -
Continuous-Flow Centrifugation to Collect Suspended Sediment for Chemical Analysis
1 Table 6. Compounds detected in both equipment blank samples and not in corresponding source blank samples or at concentrations greater than two times the corresponding source blank sample concentration. Prepared in cooperation with the National Water Quality Monitoring Council and [Source data: Appendix A, table A1; Conn and Black (2014, table A4); and Conn and others (2015, table A11). CAS Registry Number: Chemical Abstracts Service Washington State Department of(CAS) Ecology Registry Number® (RN) is a registered trademark of the American Chemical Society. CAS recommends the verifi cation of CASRNs through CAS Client ServicesSM. Method: EPA, U.S. Environmental Protection Agency’s SW 846; SIM, select ion monitoring. Unit: µg/kg, microgram per kilogram; ng/kg, nanogram per kilogram. Sample type: River samples were from the Puyallup River, Washington. Q, qualifi er (blank cells indicate an unqualifi ed detection). J, estimated, result between the Continuous-Flow Centrifugationdetection level and reporting level; toNJ, result Collect did not meet all quantitation Suspended criteria (an estimated maxiumum possible concentration is reported in Result column) U, not detected above the reporting level (reported in the Result column); UJ, not detected above the detection level (reported in the Result column). Abbreviations: na, not Sediment for Chemicalapplicable; Analysis PCBs, polychlorinated biphenyls] Sample type Chapter 6 of CAS River River Commercial Commercial Section D, Water Quality Parameter name Registry Method Unit source equipment -
State-Of-The-Art Water Treatment in Czech Power Sector
membranes Article State-of-the-Art Water Treatment in Czech Power Sector: Industry-Proven Case Studies Showing Economic and Technical Benefits of Membrane and Other Novel Technologies for Each Particular Water Cycle Jaromír Marek Department of Chemistry, Faculty of Science, Humanities and Education, Technical University of Liberec, Studentská 1402/2, 461 17 Liberec, Czech Republic; [email protected]; Tel.: +420-732-277-183 Abstract: The article first summarizes case studies on the three basic types of treated water used in power plants and heating stations. Its main focus is Czechia as the representative of Eastern European countries. Water as the working medium in the power industry presents the three most common cycles—the first is make-up water for boilers, the second is cooling water and the third is represented by a specific type of water (e.g., liquid waste mixtures, primary and secondary circuits in nuclear power plants, turbine condensate, etc.). The water treatment technologies can be summarized into four main groups—(1) filtration (coagulation) and dosing chemicals, (2) ion exchange technology, (3) membrane processes and (4) a combination of the last two. The article shows the ideal industry-proven technology for each water cycle. Case studies revealed the economic, technical and environmental advantages/disadvantages of each technology. The percentage of Citation: Marek, J. State-of-the-Art technologies operated in energetics in Eastern Europe is briefly described. Although the work is Water Treatment in Czech Power conceived as an overview of water treatment in real operation, its novelty lies in a technological model Sector: Industry-Proven Case Studies of the treatment of turbine condensate, recycling of the cooling tower blowdown plus other liquid Showing Economic and Technical waste mixtures, and the rejection of colloidal substances from the secondary circuit in nuclear power Benefits of Membrane and Other plants. -
Nanosep® Centrifugal Devices - Protocols for Use Nanosep® Centrifugal Devices - Protocols for Use
Contact Us: www.pall.com/contact Nanosep® Centrifugal Devices - Protocols for Use Nanosep® Centrifugal Devices - Protocols for Use Ultrafiltration Fundamentals Purification and Handling of DNA Fragments PCR: Before and After Protein Purification and Handling Miscellaneous Protocols Appendices References Ordering Information Ultrafiltration Fundamentals Background Ultrafiltration (UF) is a membrane separation technique used to separate extremely small particles and dissolved molecules in fluids. The primary basis for separation is molecular size, although other factors such as molecule shape and charge can also play a role. Molecules larger than the membrane pores will be retained at the surface of the membrane (not in the polymer matrix as they are retained in microporous membranes) and concentrated during the ultrafiltration process. Compared to non-membrane processes (chromatography, dialysis, solvent extraction, or centrifugation), ultrafiltration: Is far gentler to the molecules being processed. Does not require an organic extraction which may denature labile proteins. Maintains the ionic and pH milieu. Is fast and relatively inexpensive. Can be performed at low temperatures (for example, in the cold room). Is very efficient and can simultaneously concentrate and purify molecules. The retention properties of ultrafiltration membranes are expressed as Molecular Weight Cutoff (MWCO). This value refers to the approximate molecular weight (MW) of a dilute globular solute (i.e., a typical protein) which is 90% retained by the membrane. However, a molecule’s shape can have a direct effect on its retention by a membrane. For example, linear molecules like DNA may find their way through pores that will retain a globular species of the same molecular weight. There are three generic applications for ultrafiltration: 1. -
Centrifugal Hyperfiltration
TO: Technology Innovation Program Mail Stop 4750 National Institute of Standards and Technology 100 Bureau Drive Gaithersburg, MD 20899-4750 FM: Dirk Forman 125 S. St. Louis Lafayette Louisiana 70506 [email protected] Centrifugal Hyperfiltration A means to economically recover resources from saltwater and wastewater streams with lower the energy cost of producing freshwater and potable water from marginalized water resources. Introduction Reverse Osmosis (RO) is a filtration process for the removal of ionic and organic pollutants from wastewater. Today’s technology of utilization of this filtration process is by large array of high-pressure piping and pressure pumps. This process yields low volumes of filtrated output (permeate), utilizes large areas for pipe array and components and the concentration polarization and membrane fouling hinders the wide application of RO filtration process. Utilizing centrifugal forces and cross flow membranes has potential to change the standard (RO) process to one of portable and high volume water purification. AN AREA OF CRITICAL NATIONAL NEED Ensuring Future Water Supply: As the Nation’s population and economy grow, greater demands are being placed on freshwater resources. At the same time, temporary or permanent drought conditions and water access rights affect regional freshwater availability. Water needs threaten to outstrip available freshwater, now and in the future. Emerging contaminants that must either be removed from distributed water or converted to harmless forms of waste is also pressuring water quality, both in terms of decontamination and disinfection of water supplies. Food contaminations are often traced back to water contaminations, either in the field or in processing. Municipal waste streams and irrigation runoff waste resources that are not recovered.1 Over 97 percent of the Earth's water -- seawater and brackish groundwater -- is too salty to use for drinking water or agriculture. -
Removal of Waterborne Particles by Electrofiltration: Pilot-Scale Testing
ENVIRONMENTAL ENGINEERING SCIENCE Volume 26, Number 12, 2009 ª Mary Ann Liebert, Inc. DOI: 10.1089=ees.2009.0238 Removal of Waterborne Particles by Electrofiltration: Pilot-Scale Testing Ying Li,1 Ray Ehrhard,1 Pratim Biswas,1,* Pramod Kulkarni,2 Keith Carns,3 Craig Patterson,4 Radha Krishnan,5 and Rajib Sinha5 1Department of Energy, Environmental and Chemical Engineering, Washington University in St. Louis, St. Louis, Missouri. 2Center for Disease Control and Prevention, National Institute for Occupational Safety and Health, Cincinnati, Ohio. 3Global Energy Partners, LLC, Oakhurst, California. 4Office of Research and Development, National Risk Management Research Laboratory, U.S. Environmental Protection Agency, Cincinnati, Ohio. 5Shaw Environmental and Infrastructure, Inc., Cincinnati, Ohio. Received: July 6, 2009 Accepted in revised form: October 20, 2009 Abstract Theoretical analysis using a trajectory approach indicated that in the presence of an external electric field, charged waterborne particles are subject to an additional migration velocity that increases their deposition on the surface of collectors (e.g., sand filter). Although researchers conducted bench-scale experiments to verify the effectiveness of electrofiltration, few studies have reported on the applications of electrofiltration in larger scale facilities. In this study, a prototype pilot-scale electrofiltration unit, consisting of an acrylic tank (0.3Â0.3Â1.2 m) with vertically placed stainless steel mesh electrodes embedded in a sand filter was tested at a local drinking water plant. Presedimentation basin water was used as the influent with a turbidity ranging from 12 to 37 NTU. At an approach velocity of 0.84 mm=s, an electrode voltage at 8 and 12 V increased the particle removal coefficient pC* [defined as Àlog(Cout=Cin)] to 1.79 and 1.86, respectively, compared to 1.48 when there was no electric field. -
404 Sedimentation Handout
Sedimentation for Analysis and Separation of Macromolecules A ‘centrifugal force’ as experienced by particles in a centrifuge actually reflects the natural tendency of the particle to move in a straight line in the absence of external forces. If we view the experiment in the (fixed) reference frame of the laboratory, we observe that the particle is deflected from a linear trajectory by a centripetal force (directed toward the central axis of the rotor). However, when we consider the motion of the particle with respect to the (rotating) reference frame of the rotor, it appears that at any instant the particle experiences an outward or centrifugal force: Instantaneous Velocity (vtang) Centrifugal Acceleration Centripetal Acceleration As seen in laboratory As seen in (rotating) reference frame reference frame of rotor Ignoring buoyancy effects for the moment, the magnitude of the centrifugal force (which, in the rotating reference frame of the rotor, is as real as any other force) is m ⋅ v2 F = objecttan g =⋅mrω 2 centrif r object [1] where r is the distance from the axis of rotation, vtang is the linear velocity at any instant (in the direction perpendicular to the radial axis) and ω is the angular velocity (in radians sec-1 – note that 1 rpm = 2π///60 rad sec-1). The magnitude of this force can be compared to that of the gravitational force at the earth’s surface: Gm⋅()⋅() m = earth object Fgrav 2 re [2] -8 -1 3 -2 where G is the universal gravitational constant (= 6.67 x 10 g cm sec ) and re and me are the earth’s radius (= 6.37 x 108 cm) and mass (5.976 x 1027 g), respectively. -
Wastewater Treatment and Reuse in the Oil & Petrochem Industry
Engineering Conferences International ECI Digital Archives Wastewater and Biosolids Treatment and Reuse: Proceedings Bridging Modeling and Experimental Studies Spring 6-13-2014 Wastewater treatment and reuse in the oil & petrochem industry – a case study Alberto Girardi Dregemont Follow this and additional works at: http://dc.engconfintl.org/wbtr_i Part of the Environmental Engineering Commons Recommended Citation Alberto Girardi, "Wastewater treatment and reuse in the oil & petrochem industry – a case study" in "Wastewater and Biosolids Treatment and Reuse: Bridging Modeling and Experimental Studies", Dr. Domenico Santoro, Trojan Technologies and Western University Eds, ECI Symposium Series, (2014). http://dc.engconfintl.org/wbtr_i/46 This Conference Proceeding is brought to you for free and open access by the Proceedings at ECI Digital Archives. It has been accepted for inclusion in Wastewater and Biosolids Treatment and Reuse: Bridging Modeling and Experimental Studies by an authorized administrator of ECI Digital Archives. For more information, please contact [email protected]. Wastewater Treatment and Reuse In Oil & Petrochemical Industry Otranto, June 2014 COMPANY PROFILE DEGREMONT, THE WATER TREATMENT SPECIALISTS 4 areas of 5 areas of expertise: activities: . Drinking water production . Design & Build plants . Operation & . Reverse osmosis desalination Services plants . Urban wastewater treatment . Equipment and reuse plants . BOT / PPP . Biosolid treatment systems . Industrial water production and wastewater treatment units plants 2 Wastewater Treatment and Reuse COMPANY PROFILE DEGREMONT, THE WATER TREATMENT SPECIALISTS In over For industrials: For local authorities: 70 . Energy . Drinking water countries, . Upstream oil and gas Degrémont offers . Desalination . Refining and solutions to local . Urban wastewater authorities and petrochemicals . Sludge and biosolids industries . Chemicals . Pharmaceutical, cosmetics, fine chemicals . -
Guide to Gel Filtration Or Size Exclusion Chromatography
Guide to Gel Filtration or Size Exclusion Chromatography www.harvardapparatus.com Table of Contents Introduction ........................................................................ 2-4 Size Fractionation .................................................................... 5 Buffer Sample Selection ........................................................ 6-7 Selection of Media and Size .................................................... 8 Gel Filtration SpinColumns .................................................... 9 Spehadex P-25 Applications .................................................. 10 Desalting Columns Applications ............................................ 11 P-2, P-6 and P-30 SpinColumns .......................................... 12 Ordering Information ............................................................ 13 Contact Information .............................................................. 14 Guide to Gel Filtration or Size Exclusion Chromatography 1 Introduction Gel Filtration Gel Filtration also called size-exclusion chromatography can be used for protein DNA purification, buffer exchange, desalting, or for group separation in which the sample is separated in two major groups. Gel Filtration is an easy to use method for separation of molecules with different molecular sizes, using mild conditions. Gel Filtration uses the size of molecules in solution to determine separation. SpinColumns have short media packing so the samples are separated by size, the large molecules travel out of the column with the void -
Advancing Electrodeionization with Conductive Ionomer Binders That
www.nature.com/npjcleanwater ARTICLE OPEN Advancing electrodeionization with conductive ionomer binders that immobilize ion-exchange resin particles into porous wafer substrates ✉ ✉ Varada Menon Palakkal 1,3, Lauren Valentino 2,3, Qi Lei 1, Subarna Kole1, Yupo J. Lin 2 and Christopher G. Arges 1 Electrodeionization (EDI) is an electrically driven separations technology that employs ion-exchange membranes and resin particles. Deionization occurs under the influence of an applied electric field, facilitating continuous regeneration of the resins and supplementing ionic conductivity. While EDI is commercially used for ultrapure water production, material innovation is required for improving desalination performance and energy efficiency for treating alternative water supplies. This work reports a new class of ion-exchange resin-wafers (RWs) fabricated with ion-conductive binders that exhibit exceptional ionic conductivities—a3–5-fold improvement over conventional RWs that contain a non-ionic polyethylene binder. Incorporation into an EDI stack (RW-EDI) resulted in an increased desalination rate and reduced energy expenditure compared to the conventional RWs. The water-splitting phenomenon was also investigated in the RW in an external experimental setup in this work. Overall, this work demonstrates that ohmic resistances can be substantially curtailed with ionomer binder RWs at dilute salt concentrations. npj Clean Water (2020) 3:5 ; https://doi.org/10.1038/s41545-020-0052-z 1234567890():,; INTRODUCTION EDI stack is more thermodynamically efficient for removing ions in 10 Electrochemical separations, which primarily consist of electrodia- the more challenging dilute concentration regime. lysis (ED), electrodeionization (EDI), and membrane capacitive A drawback of conventional EDI is the utilization of loose resin deionization (MCDI/CDI),1 are a subset of technologies primarily beads that foster inconsistent process performance, stack leakage, used for deionization and other water treatment processes.