Determining Habitability: Which Exoearths Should We Search for Life?
Total Page:16
File Type:pdf, Size:1020Kb
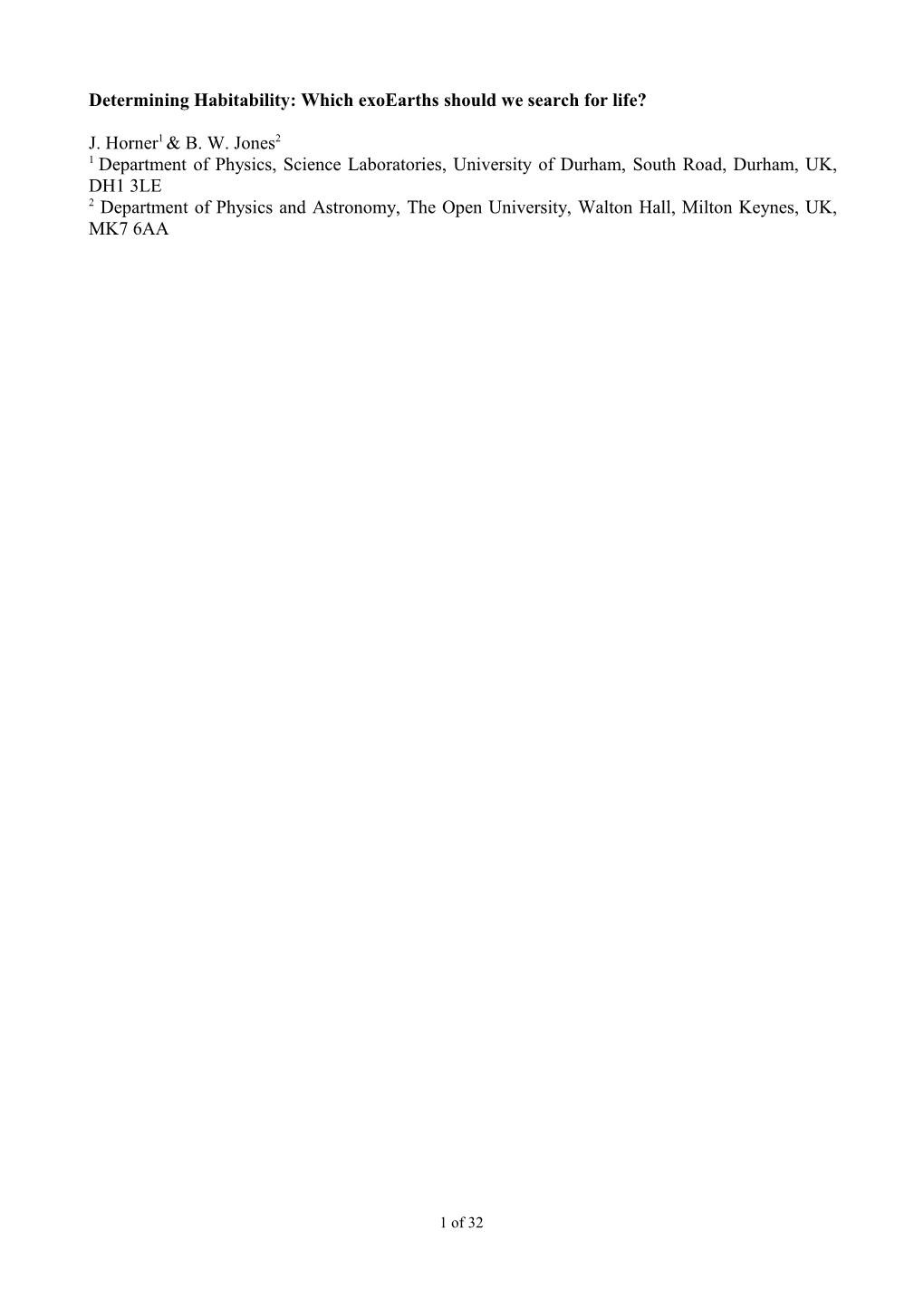
Load more
Recommended publications
-
BRAS Newsletter August 2013
www.brastro.org August 2013 Next meeting Aug 12th 7:00PM at the HRPO Dark Site Observing Dates: Primary on Aug. 3rd, Secondary on Aug. 10th Photo credit: Saturn taken on 20” OGS + Orion Starshoot - Ben Toman 1 What's in this issue: PRESIDENT'S MESSAGE....................................................................................................................3 NOTES FROM THE VICE PRESIDENT ............................................................................................4 MESSAGE FROM THE HRPO …....................................................................................................5 MONTHLY OBSERVING NOTES ....................................................................................................6 OUTREACH CHAIRPERSON’S NOTES .........................................................................................13 MEMBERSHIP APPLICATION .......................................................................................................14 2 PRESIDENT'S MESSAGE Hi Everyone, I hope you’ve been having a great Summer so far and had luck beating the heat as much as possible. The weather sure hasn’t been cooperative for observing, though! First I have a pretty cool announcement. Thanks to the efforts of club member Walt Cooney, there are 5 newly named asteroids in the sky. (53256) Sinitiere - Named for former BRAS Treasurer Bob Sinitiere (74439) Brenden - Named for founding member Craig Brenden (85878) Guzik - Named for LSU professor T. Greg Guzik (101722) Pursell - Named for founding member Wally Pursell -
I N S I D E T H I S I S S
Publications from February/février 1998 Volume/volume 92 Number/numero 1 [669] The Royal Astronomical Society of Canada The Beginner’s Observing Guide This guide is for anyone with little or no experience in observing the night sky. Large, easy to read star maps are provided to acquaint the reader with the constellations and bright stars. Basic information on observing the moon, planets and eclipses through the year 2000 is provided. There The Journal of the Royal Astronomical Society of Canada Le Journal de la Société royale d’astronomie du Canada is also a special section to help Scouts, Cubs, Guides and Brownies achieve their respective astronomy badges. Written by Leo Enright (160 pages of information in a soft-cover book with a spiral binding which allows the book to lie flat). Price: $12 (includes taxes, postage and handling) Looking Up: A History of the Royal Astronomical Society of Canada Published to commemorate the 125th anniversary of the first meeting of the Toronto Astronomical Club, “Looking Up — A History of the RASC” is an excellent overall history of Canada’s national astronomy organization. The book was written by R. Peter Broughton, a Past President and expert on the history of astronomy in Canada. Histories on each of the centres across the country are included as well as dozens of biographical sketches of the many people who have volunteered their time and skills to the Society. (hard cover with cloth binding, 300 pages with 150 b&w illustrations) Price: $43 (includes taxes, postage and handling) Observers Calendar — 1998 This calendar was created by members of the RASC. -
The Denver Observer December 2017
The Denver DECEMBER 2017 OBSERVER Messier 76, the Little Dumbbell Nebula, one of the deep-sky objects featured in this month’s “Skies.” Image © Joe Gafford. DECEMBER SKIES by Zachary Singer The Solar System of view in your ’scope will include the Moon’s Sky Calendar 3 Full Moon December will be a decent month for eastern section and the star, with plenty of 10 Last-Quarter Moon planetary events; though some planets are room. 17 New Moon slipping from view, others will take their I recommend you observe early—it 26 First-Quarter Moon place. We also have an occultation of Alde- should be a beautiful view, with the star a baran; as seen from Denver, the Moon will bright spark near the Moon’s edge, and over pass in front of the star at approximately the following minutes (they’ll go fast, just like In the Observer 4:06 PM, on the 30th. At that point, with the recent solar eclipse did), you can see the Moon move in its orbit around us, using the sunset still more than half an hour away, the President’s Message . .2 star won’t be visible to the naked eye, but it star for a benchmark. (Before 4:00 PM, look Society Directory. 2 should be in a telescope if you know where for Aldebaran outside the square, but along to look: Imagine a square drawn just large the diagonal from the Moon’s center to that Schedule of Events . 2 enough to touch the edges of the Moon, and lower-left edge.) About Denver Astronomical Society . -
Musical Composition Graduate Portfolio
University of Northern Iowa UNI ScholarWorks Dissertations and Theses @ UNI Student Work 2021 Musical composition graduate portfolio Juan Marulanda University of Northern Iowa Let us know how access to this document benefits ouy Copyright ©2021 Juan Marulanda Follow this and additional works at: https://scholarworks.uni.edu/etd Recommended Citation Marulanda, Juan, "Musical composition graduate portfolio" (2021). Dissertations and Theses @ UNI. 1102. https://scholarworks.uni.edu/etd/1102 This Open Access Thesis is brought to you for free and open access by the Student Work at UNI ScholarWorks. It has been accepted for inclusion in Dissertations and Theses @ UNI by an authorized administrator of UNI ScholarWorks. For more information, please contact [email protected]. Copyright by JUAN MARULANDA 2021 All Rights Reserved MUSICAL COMPOSITION GRADUATE PORTFOLIO An Abstract Submitted in Partial Fulfillment of the Requirements for the Degree Master of Music Juan Marulanda University of Northern Iowa May 2021 This Study By: Juan Carlos Marulanda Entitled: Musical Composition Graduate Portfolio has been approved as meeting the thesis requirement for the Degree of Master of Music: Composition Date Dr. Daniel Swilley, Chair, Recital Committee Date Dr. Michael Conrad, Recital Committee Member Date Dr. Jonathan Schwabe, Recital Committee Member Date Dr. Jennifer Waldron, Dean, Graduate College This Recital Performance By: Juan Marulanda Entitled: Musical Composition Graduate Portfolio has been approved as meeting the thesis requirement for the Degree of Master of Music: Composition Date Dr. Daniel Swilley, Chair, Recital Committee Date Dr. Michael Conrad, Recital Committee Member Date Dr. Jonathan Schwabe, Recital Committee Member Date Dr. Jennifer Waldron, Dean, Graduate College ABSTRACT The musical works included in this portfolio were composed between Fall 2019 and Spring 2021. -
Vssc163 Draftv3 IBVS 2 Colour Correct Graph.Pmd
British Astronomical Association VARIABLE STAR SECTION CIRCULAR No 163, March 2015 Contents IBVS 6080 – 6109 - J. Simpson ............................................... inside front cover From the Director - R. Pickard ........................................................................... 3 Polar V1432 Aquilae - Editor’s Note .................................................................. 4 Eclipsing Binary News - D. Loughney .............................................................. 4 Update on the Campaign to Observe the Dwarf Nova CSS 121005:212625+201948 - J. Shears ............... 6 How Variable Stars get Their Names - D. Griffin .............................................. 9 References to Naming of Variable Stars - D. Griffin ........................................ 11 The Discovery of Three New Variable Stars using the Bradford Robotic Telescope and the Software Package Muniwin - D. Conner .................. 12 FY Librae - a First Look at the Behaviour during 2014 - P. Williams .............. 16 FY Librae goes Active and Reaffirms the Howarth and Bailey Formula - J. Toone .............. 18 Refining the Period of V505 Scuti - I. Miller ................................................... 21 Binocular Programme - M. Taylor ................................................................... 21 Eclipsing Binary Predictions – Where to Find Them - D. Loughney .............. 22 Charges for Section Publications .............................................. inside back cover Guidelines for Contributing to the Circular ............................. -
Biosignatures Search in Habitable Planets
galaxies Review Biosignatures Search in Habitable Planets Riccardo Claudi 1,* and Eleonora Alei 1,2 1 INAF-Astronomical Observatory of Padova, Vicolo Osservatorio, 5, 35122 Padova, Italy 2 Physics and Astronomy Department, Padova University, 35131 Padova, Italy * Correspondence: [email protected] Received: 2 August 2019; Accepted: 25 September 2019; Published: 29 September 2019 Abstract: The search for life has had a new enthusiastic restart in the last two decades thanks to the large number of new worlds discovered. The about 4100 exoplanets found so far, show a large diversity of planets, from hot giants to rocky planets orbiting small and cold stars. Most of them are very different from those of the Solar System and one of the striking case is that of the super-Earths, rocky planets with masses ranging between 1 and 10 M⊕ with dimensions up to twice those of Earth. In the right environment, these planets could be the cradle of alien life that could modify the chemical composition of their atmospheres. So, the search for life signatures requires as the first step the knowledge of planet atmospheres, the main objective of future exoplanetary space explorations. Indeed, the quest for the determination of the chemical composition of those planetary atmospheres rises also more general interest than that given by the mere directory of the atmospheric compounds. It opens out to the more general speculation on what such detection might tell us about the presence of life on those planets. As, for now, we have only one example of life in the universe, we are bound to study terrestrial organisms to assess possibilities of life on other planets and guide our search for possible extinct or extant life on other planetary bodies. -
Correlations Between the Stellar, Planetary, and Debris Components of Exoplanet Systems Observed by Herschel⋆
A&A 565, A15 (2014) Astronomy DOI: 10.1051/0004-6361/201323058 & c ESO 2014 Astrophysics Correlations between the stellar, planetary, and debris components of exoplanet systems observed by Herschel J. P. Marshall1,2, A. Moro-Martín3,4, C. Eiroa1, G. Kennedy5,A.Mora6, B. Sibthorpe7, J.-F. Lestrade8, J. Maldonado1,9, J. Sanz-Forcada10,M.C.Wyatt5,B.Matthews11,12,J.Horner2,13,14, B. Montesinos10,G.Bryden15, C. del Burgo16,J.S.Greaves17,R.J.Ivison18,19, G. Meeus1, G. Olofsson20, G. L. Pilbratt21, and G. J. White22,23 (Affiliations can be found after the references) Received 15 November 2013 / Accepted 6 March 2014 ABSTRACT Context. Stars form surrounded by gas- and dust-rich protoplanetary discs. Generally, these discs dissipate over a few (3–10) Myr, leaving a faint tenuous debris disc composed of second-generation dust produced by the attrition of larger bodies formed in the protoplanetary disc. Giant planets detected in radial velocity and transit surveys of main-sequence stars also form within the protoplanetary disc, whilst super-Earths now detectable may form once the gas has dissipated. Our own solar system, with its eight planets and two debris belts, is a prime example of an end state of this process. Aims. The Herschel DEBRIS, DUNES, and GT programmes observed 37 exoplanet host stars within 25 pc at 70, 100, and 160 μm with the sensitiv- ity to detect far-infrared excess emission at flux density levels only an order of magnitude greater than that of the solar system’s Edgeworth-Kuiper belt. Here we present an analysis of that sample, using it to more accurately determine the (possible) level of dust emission from these exoplanet host stars and thereafter determine the links between the various components of these exoplanetary systems through statistical analysis. -
The Maunder Minimum and the Variable Sun-Earth Connection
The Maunder Minimum and the Variable Sun-Earth Connection (Front illustration: the Sun without spots, July 27, 1954) By Willie Wei-Hock Soon and Steven H. Yaskell To Soon Gim-Chuan, Chua Chiew-See, Pham Than (Lien+Van’s mother) and Ulla and Anna In Memory of Miriam Fuchs (baba Gil’s mother)---W.H.S. In Memory of Andrew Hoff---S.H.Y. To interrupt His Yellow Plan The Sun does not allow Caprices of the Atmosphere – And even when the Snow Heaves Balls of Specks, like Vicious Boy Directly in His Eye – Does not so much as turn His Head Busy with Majesty – ‘Tis His to stimulate the Earth And magnetize the Sea - And bind Astronomy, in place, Yet Any passing by Would deem Ourselves – the busier As the Minutest Bee That rides – emits a Thunder – A Bomb – to justify Emily Dickinson (poem 224. c. 1862) Since people are by nature poorly equipped to register any but short-term changes, it is not surprising that we fail to notice slower changes in either climate or the sun. John A. Eddy, The New Solar Physics (1977-78) Foreword By E. N. Parker In this time of global warming we are impelled by both the anticipated dire consequences and by scientific curiosity to investigate the factors that drive the climate. Climate has fluctuated strongly and abruptly in the past, with ice ages and interglacial warming as the long term extremes. Historical research in the last decades has shown short term climatic transients to be a frequent occurrence, often imposing disastrous hardship on the afflicted human populations. -
Exoplanet.Eu Catalog Page 1 # Name Mass Star Name
exoplanet.eu_catalog # name mass star_name star_distance star_mass OGLE-2016-BLG-1469L b 13.6 OGLE-2016-BLG-1469L 4500.0 0.048 11 Com b 19.4 11 Com 110.6 2.7 11 Oph b 21 11 Oph 145.0 0.0162 11 UMi b 10.5 11 UMi 119.5 1.8 14 And b 5.33 14 And 76.4 2.2 14 Her b 4.64 14 Her 18.1 0.9 16 Cyg B b 1.68 16 Cyg B 21.4 1.01 18 Del b 10.3 18 Del 73.1 2.3 1RXS 1609 b 14 1RXS1609 145.0 0.73 1SWASP J1407 b 20 1SWASP J1407 133.0 0.9 24 Sex b 1.99 24 Sex 74.8 1.54 24 Sex c 0.86 24 Sex 74.8 1.54 2M 0103-55 (AB) b 13 2M 0103-55 (AB) 47.2 0.4 2M 0122-24 b 20 2M 0122-24 36.0 0.4 2M 0219-39 b 13.9 2M 0219-39 39.4 0.11 2M 0441+23 b 7.5 2M 0441+23 140.0 0.02 2M 0746+20 b 30 2M 0746+20 12.2 0.12 2M 1207-39 24 2M 1207-39 52.4 0.025 2M 1207-39 b 4 2M 1207-39 52.4 0.025 2M 1938+46 b 1.9 2M 1938+46 0.6 2M 2140+16 b 20 2M 2140+16 25.0 0.08 2M 2206-20 b 30 2M 2206-20 26.7 0.13 2M 2236+4751 b 12.5 2M 2236+4751 63.0 0.6 2M J2126-81 b 13.3 TYC 9486-927-1 24.8 0.4 2MASS J11193254 AB 3.7 2MASS J11193254 AB 2MASS J1450-7841 A 40 2MASS J1450-7841 A 75.0 0.04 2MASS J1450-7841 B 40 2MASS J1450-7841 B 75.0 0.04 2MASS J2250+2325 b 30 2MASS J2250+2325 41.5 30 Ari B b 9.88 30 Ari B 39.4 1.22 38 Vir b 4.51 38 Vir 1.18 4 Uma b 7.1 4 Uma 78.5 1.234 42 Dra b 3.88 42 Dra 97.3 0.98 47 Uma b 2.53 47 Uma 14.0 1.03 47 Uma c 0.54 47 Uma 14.0 1.03 47 Uma d 1.64 47 Uma 14.0 1.03 51 Eri b 9.1 51 Eri 29.4 1.75 51 Peg b 0.47 51 Peg 14.7 1.11 55 Cnc b 0.84 55 Cnc 12.3 0.905 55 Cnc c 0.1784 55 Cnc 12.3 0.905 55 Cnc d 3.86 55 Cnc 12.3 0.905 55 Cnc e 0.02547 55 Cnc 12.3 0.905 55 Cnc f 0.1479 55 -
Ghost Imaging of Space Objects
Ghost Imaging of Space Objects Dmitry V. Strekalov, Baris I. Erkmen, Igor Kulikov, and Nan Yu Jet Propulsion Laboratory, California Institute of Technology, 4800 Oak Grove Drive, Pasadena, California 91109-8099 USA NIAC Final Report September 2014 Contents I. The proposed research 1 A. Origins and motivation of this research 1 B. Proposed approach in a nutshell 3 C. Proposed approach in the context of modern astronomy 7 D. Perceived benefits and perspectives 12 II. Phase I goals and accomplishments 18 A. Introducing the theoretical model 19 B. A Gaussian absorber 28 C. Unbalanced arms configuration 32 D. Phase I summary 34 III. Phase II goals and accomplishments 37 A. Advanced theoretical analysis 38 B. On observability of a shadow gradient 47 C. Signal-to-noise ratio 49 D. From detection to imaging 59 E. Experimental demonstration 72 F. On observation of phase objects 86 IV. Dissemination and outreach 90 V. Conclusion 92 References 95 1 I. THE PROPOSED RESEARCH The NIAC Ghost Imaging of Space Objects research program has been carried out at the Jet Propulsion Laboratory, Caltech. The program consisted of Phase I (October 2011 to September 2012) and Phase II (October 2012 to September 2014). The research team consisted of Drs. Dmitry Strekalov (PI), Baris Erkmen, Igor Kulikov and Nan Yu. The team members acknowledge stimulating discussions with Drs. Leonidas Moustakas, Andrew Shapiro-Scharlotta, Victor Vilnrotter, Michael Werner and Paul Goldsmith of JPL; Maria Chekhova and Timur Iskhakov of Max Plank Institute for Physics of Light, Erlangen; Paul Nu˜nez of Coll`ege de France & Observatoire de la Cˆote d’Azur; and technical support from Victor White and Pierre Echternach of JPL. -
Universidade De São Paulo Instituto De Astronomia E Geofisica Mestrado Profissional Em Ensino De Astronomia
UNIVERSIDADE DE SÃO PAULO INSTITUTO DE ASTRONOMIA E GEOFISICA MESTRADO PROFISSIONAL EM ENSINO DE ASTRONOMIA JOSÉ IVAN SPINARDI ELABORAÇÃO DE UMA SEQUÊNCIA DIDÁTICA EM ASTROBIOLOGIA PARA O ENSINO FUNDAMENTAL 2 SÃO PAULO 2017 “Versão corrigida. O original encontra-se disponível na Unidade.” JOSÉ IVAN SPINARDI ELABORAÇÃO DE UMA SEQUÊNCIA DIDÁTICA EM ASTROBIOLOGIA PARA O ENSINO FUNDAMENTAL 2 Dissertação apresentada ao Instituto de Astronomia e Geofísica da Universidade de São Paulo obtenção do título de mestre em ensino de Astronomia. Área de concentração: Ensino de Astronomia. Orientador: Prof. Dr. Amâncio Cesar Santos Friaça SÃO PAULO 2017 “O materialismo dialético ensina que a matéria nunca permanece em repouso, mas, pelo contrário, está em constante movimento, desenvolve-se e, evoluindo, eleva-se a níveis cada vez mais altos e adquire formas de movimento cada vez mais complexas. Ao elevar-se de um degrau ao outro, a matéria adquire novos atributos. Um deles é a vida cujo surgimento marca uma etapa, um escalão determinado do desenvolvimento histórico da matéria. Durante o decorrer desse desenvolvimento é que surgiu a vida, como uma nova qualidade.” Alexander Oparin “Se não existe vida fora da Terra, então o Universo é um grande desperdício de espaço” Carl Sagan “Há vidas sim em outros planetas, porém, não desenvolvidas.” Um aluno da 8ª série A. AGRADECIMENTOS À minha família. Ao meu sobrinho Luiz Lucas pelo suporte em informática. Às educadoras Erika Reyes Molina e Giovanni Scataglia Botelho Paz, pelo apoio e confiança. À Professora Maria Helena Pereira, da EMEF João Guimaraes Rosa, pela cessão de suas aulas para a aplicação das atividades. -
Hunting for Transits of Super-Earth GJ 581E 2 June 2011
Hunting for transits of Super-Earth GJ 581e 2 June 2011 terrestrial-mass exoplanet in the habitable zone". They found that, despite the small amount of light Gliese 581d receives from its star, a carbon dioxide atmosphere could maintain temperatures above the freezing point of water at the surface. If the orbital inclination of a planetary system is just right, then one or more planets may be observed to cross (transit) the disk of the star, dimming the brightness of the star by a tiny fraction. By measuring this effect, it is possible to derive the Artist's concept of Gliese 581 e. Image: size of the planet. JohnVanVliet/Wikipedia. If Gliese 581e transits its host star, then it must have a radius smaller than 1.4 times that of the Earth since a larger planet would have been (PhysOrg.com) -- An international team of detected by MOST. In terms of composition, this astronomers has ruled out transits of a water-rich suggests that planet e does not have a hydrogen or or hydrogen-helium atmosphere planet for Gliese helium atmosphere, nor does it contain more than 581e. The host star itself is relatively quiet which 25% water. Therefore it must be a solid rocky means good news for the potential habitability of at planet. However, if the planet does not transit, its least one of its planets. size and therefore its composition cannot be constrained, but the inclination of its orbit can. The study was conducted using observations acquired by MOST (Microvariability & Oscillations "Although transits were not detected for Gliese of STars), Canada's only space telescope.