Detection of F1 Hybrids from Single-Genome Data Reveals Frequent Hybridization in Hymenoptera and Particularly Ants
Total Page:16
File Type:pdf, Size:1020Kb
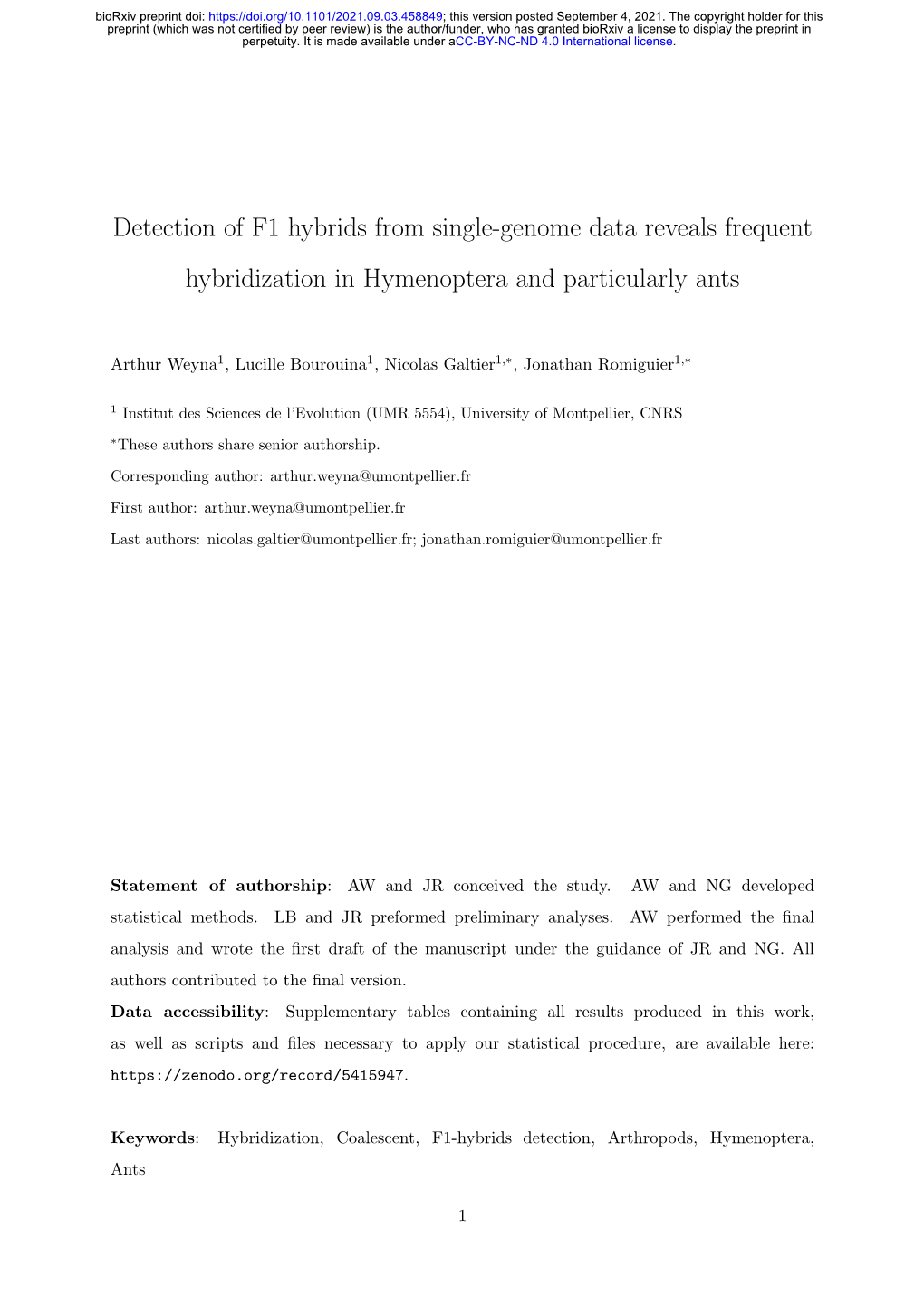
Load more
Recommended publications
-
Myrmicinae: Crematogastrini] Dacatria Rigato, 1994B: 155
DACATRIA [Myrmicinae: Crematogastrini] Dacatria Rigato, 1994b: 155. Type-species: Dacatria templaris Rigato, 1994b: 157, by original designation. Taxonomic history Dacatria in Myrmicinae, Proattini: Rigato, 1994b: 161. Dacatria in Myrmicinae, Stenammini: Bolton, 1994: 106; Bolton, 1995b: 26; Bolton, 2003: 59, 203. Dacatria in Myrmicinae, Crematogastrini: Ward, et al. 2015: 77; in Crematogastrini, Mayriella genus group: Blaimer, et al. 2018: 7. Dacatria as genus: all authors. Dacatria references: Eguchi, et al. 2011: 15 (diagnosis, Vietnam synopsis). DACETINOPS [Myrmicinae: Crematogastrini] Dacetinops Brown & Wilson, 1957a: 1. Type-species: Dacetinops cibdela Brown & Wilson, 1957: 4, by original designation. Taxonomic history Dacetinops in Myrmicinae, Leptothoracini: Wheeler, G.C. & Wheeler, J. 1985: 257. Dacetinops incertae sedis in Myrmicinae: Dlussky & Fedoseeva, 1988: 80. Dacetinops in Myrmicinae, Stenammini: Bolton, 1994: 106; Bolton, 1995b: 26; Bolton, 2003: 59, 203. Dacetinops in Myrmicinae, Crematogastrini: Ward, et al. 2015: 77; in Crematogastrini, Vollenhovia genus group: Blaimer, et al. 2018: 7. Dacetinops as genus: all authors. Dacetinops catalogues: Bolton, 1995b: 168. Dacetinops references: Taylor, 1985: 49 (all species revision, key). DACETON [Myrmicinae: Attini] Daceton Perty, 1833: 136. Type-species: Formica armigera Latreille, 1802c: 244, by monotypy. Taxonomic history Daceton in Poneridae, Myrmicidae: Smith, F. 1858b: 160. Daceton in Myrmicidae, Cryptoceridae: Smith, F. 1853: 226; Emery, 1877a: 81. Daceton in Cryptoceridae, Dacetini: Ashmead, 1905b: 384 [Dacetonini]. Daceton in Myrmicinae: Mayr, 1865: 26 [Myrmicidae]; Dalla Torre, 1893: 149 [Myrmicinae]. Daceton in Myrmicinae, Dacetini, Dacetiti: Brown, 1952g: 10 (footnote); Brown, 1954b: 465; Brown & Wilson, 1959b: 281; Brandão, 1991: 391. Daceton in Myrmicinae, Dacetini: Forel, 1893a: 164; Forel, 1892d: 344; Forel, 1895b: 136; Emery, 1895j: 770; Emery, 1896e: 180; Wheeler, W.M. -
Global Generic Richness and Distribution: New Maps of the World of Ants with Examples of Their Use in the Context of Asia
ASIAN MYRMECOLOGY Volume 3, 21–28, 2010 ISSN 1985-1944 ©BENOIT GUÉNARD, MICHAEL D. WEISER AND ROBERT R. DUNN Global generic richness and distribution: new maps of the world of ants with examples of their use in the context of Asia BENOIT GUÉNARD, MICHAEL D. WEISER AND ROBERT R. DUNN Department of Biology, North Carolina State University, Raleigh, NC, 27607, USA ABSTRACT. Knowledge of the biogeographic distribution of ants is central to our understanding of ant ecology, evolution, taxonomy and conservation. Here, we introduce a novel global biogeographic database for ant genera and an associated website with maps showing the known distribution of all extant ant genera. We use this database to consider knowledge of the distribution of ant genera in Asia, a hotspot of ant diversity and biological diversity more generally. We find that, although ant systematists and ecologists are now active in Asia, much remains to be learned about the distribution of Asian ant genera. We highlight areas where additional research would be particularly useful. In Asia, as elsewhere, ants are ecologically In this article, we describe briefly a new dominant and conspicuous actors in most resource for myrmecologists: online maps of the ecosystems: as predators (Steghaus-Kovac & known distribution of all extant ant genera. We Maschwitz 1993; Berghoff et al. 2002), mutualist focus, in this first paper, on Asia, because it is not partners with other insects (Way 1963; Maschwitz only a region of very active ant research and high & Hänel 1985; Pierce et al. 2002; Pfeiffer & generic richness (Fisher 2009), but also because Linsenmair 2007) or plants (Fiala et al. -
Composition of Canopy Ants (Hymenoptera: Formicidae) at Ton Nga Chang Wildlife Sanctuary, Songkhla Province, Thailand
ORIGINAL ARTICLE Composition of canopy ants (Hymenoptera: Formicidae) at Ton Nga Chang Wildlife Sanctuary, Songkhla Province, Thailand Suparoek Watanasit1, Surachai Tongjerm2 and Decha Wiwatwitaya3 Abstract Watanasit, S., Tongjerm, S. and Wiwatwitaya, D. Composition of canopy ants (Hymenoptera: Formicidae) at Ton Nga Chang Wildlife Sanctuary, Songkhla Province, Thailand Songklanakarin J. Sci. Technol., Dec. 2005, 27(Suppl. 3) : 665-673 Canopy ants were examined in terms of a number of species and species composition between in high and low disturbance sites of lowland tropical rainforest at Ton Nga Chang Wildlife Sanctuary, Songkhla province, Thailand, from November 2001 to November 2002. A permanent plot of 100x100 m2 was set up and divided into 100 sub-units (10x10m2) on each study site. Pyrethroid fogging was two monthly applied to collect ants on three trees at random in a permanent plot. A total of 118 morphospecies in 29 genera belonging to six subfamilies were identified. The Formicinae subfamily found the highest species numbers (64 species) followed by Myrmicinae (32 species), Pseudomyrmecinae (10 species), Ponerinae (6 species), Dolichoderinae (5 species) and Aenictinae (1 species). Myrmicinae and Ponerinae showed a significant difference of mean species number between sites (P<0.05) while Formicinae and Myrmicinae also showed a significant difference of mean species number between months (P<0.05). However, there were no interactions between sites and months in any subfamily. Key words : ants, canopy, species composition, distrubance, Songkhla, Thailand 1M.Sc.(Zoology), Assoc. Prof. 2M.Sc. Student in Biology, Department of Biology, Faculty of Science, Prince of Songkla University, Hat Yai, Songkhla 90112 Thailand. 3D.Agr., Department of Forest Biology, Faculty of Forestry, Kasetsart University, Chatuchak, Bangkok 10900 Thailand. -
Stuttgarter Beiträge Zur Naturkunde Serie B (Geologie Und Paläontologie)
Stuttgarter Beiträge zur Naturkunde Serie B (Geologie und Paläontologie) Herausgeber: Staatliches Museum für Naturkunde, Rosenstein 1, D-70191 Stuttgart Stuttgarter Beitr. Naturk. Ser. B Nr. 306 58 pp., 56 figs. Stuttgart, 31. 8. 2001 New parasitic wasps from Baltic amber (Insecta: Hymenoptera: Dryinidae) By Massimo Olmi, Viterbo and Günter Bechly, Stuttgart With 56 Figures Summary Four new species of the parasitic wasp family Dryinidae, Dryinus reifi n. sp., Dryinus muenchi n. sp., Dryinus wunderlichi n. sp., and Palaeodryinus groehni n. gen. n. sp., are de- scribed from Baltic amber. These new species belong to the widespread and diverse subfami- ly Dryininae. A neotype is designated for Harpactosphecion gracile (BRUES, 1933b) comb. nov. which was originally described in the extant genus Thaumatodryinus and is here trans- ferred to the fossil genus Harpactosphecion within Dryininae, just like Harpactosphecion? deletum (BRUES, 1933b) comb. nov. The spelling of the names of the species here attributed to the genus Harpactosphecion is changed to gracile, deletum, filicorne, and sucinum in accor- dance with Art. 30 IRZN. New specimens of Dryinus bruesi (OLMI, 1984), Dryinus janzeni OLMI, 1999b, Harpactosphecion filicorne (BRUES, 1923), and Palaeoanteon janzeni OLMI, 1999b are figured, and a new subfamily Palaeoantoninae n. subf. is established because of the new evidence. The fossil subfamily Laberitinae OLMI, 1989 is regarded as unavailable taxon since the type genus Laberites PONOMARENKO, 1988 is not available either. Updated keys to the subfamilies of Dryinidae, the genera of Dryininae, and all dryinid species from Baltic am- ber are provided, as well as an annotated catalogue of all fossil Dryinidae. Zusammenfassung Vier neue Arten der parasitischen Wespenfamilie Dryinidae, Dryinus reifi n. -
Radiation in Socially Parasitic Formicoxenine Ants
RADIATION IN SOCIALLY PARASITIC FORMICOXENINE ANTS DISSERTATION ZUR ERLANGUNG DES DOKTORGRADES DER NATURWISSENSCHAFTEN (D R. R ER . N AT .) DER NATURWISSENSCHAFTLICHEN FAKULTÄT III – BIOLOGIE UND VORKLINISCHE MEDIZIN DER UNIVERSITÄT REGENSBURG vorgelegt von Jeanette Beibl aus Landshut 04/2007 General Introduction II Promotionsgesuch eingereicht am: 19.04.2007 Die Arbeit wurde angeleitet von: Prof. Dr. J. Heinze Prüfungsausschuss: Vorsitzender: Prof. Dr. S. Schneuwly 1. Prüfer: Prof. Dr. J. Heinze 2. Prüfer: Prof. Dr. S. Foitzik 3. Prüfer: Prof. Dr. P. Poschlod General Introduction I TABLE OF CONTENTS GENERAL INTRODUCTION 1 CHAPTER 1: Six origins of slavery in formicoxenine ants 13 Introduction 15 Material and Methods 17 Results 20 Discussion 23 CHAPTER 2: Phylogeny and phylogeography of the Mediterranean species of the parasitic ant genus Chalepoxenus and its Temnothorax hosts 27 Introduction 29 Material and Methods 31 Results 36 Discussion 43 CHAPTER 3: Phylogenetic analyses of the parasitic ant genus Myrmoxenus 46 Introduction 48 Material and Methods 50 Results 54 Discussion 59 CHAPTER 4: Cuticular profiles and mating preference in a slave-making ant 61 Introduction 63 Material and Methods 65 Results 69 Discussion 75 CHAPTER 5: Influence of the slaves on the cuticular profile of the slave-making ant Chalepoxenus muellerianus and vice versa 78 Introduction 80 Material and Methods 82 Results 86 Discussion 89 GENERAL DISCUSSION 91 SUMMARY 99 ZUSAMMENFASSUNG 101 REFERENCES 103 APPENDIX 119 DANKSAGUNG 120 General Introduction 1 GENERAL INTRODUCTION Parasitism is an extremely successful mode of life and is considered to be one of the most potent forces in evolution. As many degrees of symbiosis, a phenomenon in which two unrelated organisms coexist over a prolonged period of time while depending on each other, occur, it is not easy to unequivocally define parasitism (Cheng, 1991). -
Somerset's Ecological Network
Somerset’s Ecological Network Mapping the components of the ecological network in Somerset 2015 Report This report was produced by Michele Bowe, Eleanor Higginson, Jake Chant and Michelle Osbourn of Somerset Wildlife Trust, and Larry Burrows of Somerset County Council, with the support of Dr Kevin Watts of Forest Research. The BEETLE least-cost network model used to produce Somerset’s Ecological Network was developed by Forest Research (Watts et al, 2010). GIS data and mapping was produced with the support of Somerset Environmental Records Centre and First Ecology Somerset Wildlife Trust 34 Wellington Road Taunton TA1 5AW 01823 652 400 Email: [email protected] somersetwildlife.org Front Cover: Broadleaved woodland ecological network in East Mendip Contents 1. Introduction .................................................................................................................... 1 2. Policy and Legislative Background to Ecological Networks ............................................ 3 Introduction ............................................................................................................... 3 Government White Paper on the Natural Environment .............................................. 3 National Planning Policy Framework ......................................................................... 3 The Habitats and Birds Directives ............................................................................. 4 The Conservation of Habitats and Species Regulations 2010 .................................. -
Ants of the Genus Lordomyrma Emery (2) the Japanese L. Azumai
Zootaxa 3282: 45–60 (2012) ISSN 1175-5326 (print edition) www.mapress.com/zootaxa/ Article ZOOTAXA Copyright © 2012 · Magnolia Press ISSN 1175-5334 (online edition) Ants of the genus Lordomyrma Emery (2) The Japanese L. azumai (Santschi) and six new species from India, Viet Nam and the Philippines (Hymenoptera: Formicidae: Myrmicinae) ROBERT W. TAYLOR Research School of Biological Sciences, Australian National University, Canberra, ACT, 2600, Australia. Honorary Research Fellow, CSIRO Ecosystem Sciences, Canberra, ACT, 2601, Australia. E-mail: [email protected] Abstract Lordomyrma is recorded for the first time from India and mainland southeast Asia. The Japanese L. azumai is reviewed and six new worker-based species described: L. lakshmi (Kerala State, India); L. hmong (Lao Cai Province, Vietnam); L. diwata, L. emarginata and L. idianale (Mt Isarog, Luzon, Philippines) and L. limatula (Leyte, Philippines). Gynes are characterized for L. azumai, L. hmong and L. limatula. All taxa are diagnosed, illustrated, and their affinities discussed. Key words: Ants, Formicidae, Lordomyrma, Stenamma, Lasiomyrma, taxonomy, new species, biogeography, Japan, Hon- shu, Shikoku, Kyushu, India, Kerala, Viet Nam, Lao Cai, Philippines, Luzon, Leyte, Mt Isarog Introduction This is the second paper (following Taylor, 2009) of a project seeking to review and name the many known undescribed morphospecies reasonably considered taxonomically congeneric with the somewhat aberrant ant Lordomyrma furcifera Emery (type-species of Lordomyrma Emery 1897) and its more conservative putative relative L. azumai (Santschi). The latter is arguably the least morphologically derived known Lordomyrma species (Taylor, 2009) and its characteristics may thus be very generally considered archetypal for the genus. In this analysis L. -
Ants of the Genera Myopias and Acanthoponera.1
PSYCHE VOL. XXX. DECEMBER 1923 No. 6 ANTS OF THE GENERA MYOPIAS AND ACANTHOPONERA.1 WILLIAM MORTON WHEELER. A recent study of the Australian ants collected some years ago by Mr. A. M. Lee and myself has led me to revise the Pone- .fine genera Myopias and Acanthoponera, two groups of more than usual interest on account of their singular geographical distribution. The former genus was established by Roger more than 60 years ago for a Ceylonese ant, M. amblyops, which has not been taken since, although considerable thorough collecting has been done in India and Ceylon. A second species was brought to light in New Guinea by L. Bir6 and described in 1901 by Emery as M. cribriceps. A third species has now been discovered by Mr. Lea in Tasmania and is described in the sequel. The highly vestigial eyes in the workers of these ants show that they are subterranean in habit, but they must be extremely rare, since a total of only eleven specimens has been seen. Their recorded distribution is so discontinuous that we may regard them as vanishing relicts of forms very close to the direct ancestors of Trapeziopelta, a genus represented by a number of species in the East Indies and New Guinea. The distribution of Acanthoponera is even more interesting. It comprises two species in Australia, one in New Zealand and five in the Neotropical Region, from Chile, Argentina and Brazil to Central America and Mexico. All the American specms occur in the southern portion of the range and the forms in Central America and Mexico are merely small varieties or subspecies which have strayed beyond the optimum environ- 1Contributions from the Entomological Laboratory of the Bussey Insti- tution. -
Redalyc.A Checklist of the Ants (Hymenoptera: Formicidae) of The
Agronomía Colombiana ISSN: 0120-9965 [email protected] Universidad Nacional de Colombia Colombia Vergara-Navarro, Erika Valentina; Serna, Francisco A checklist of the ants (Hymenoptera: Formicidae) of the department of Antioquia, Colombia and new records for the country Agronomía Colombiana, vol. 31, núm. 3, 2013, pp. 324-342 Universidad Nacional de Colombia Bogotá, Colombia Available in: http://www.redalyc.org/articulo.oa?id=180329804008 How to cite Complete issue Scientific Information System More information about this article Network of Scientific Journals from Latin America, the Caribbean, Spain and Portugal Journal's homepage in redalyc.org Non-profit academic project, developed under the open access initiative CROP PROTECTION A checklist of the ants (Hymenoptera: Formicidae) of the department of Antioquia, Colombia and new records for the country Lista de las hormigas (Hymenoptera: Formicidae) del departamento de Antioquia, Colombia, y nuevos registros para el país Erika Valentina Vergara-Navarro1, 2 and Francisco Serna2, 3 ABSTRACT RESUMEN Antioquia is a state (department) of Colombia, located in the Antioquia es un departamento de Colombia localizado en los northwestern Andes of South America. Geologically, the north- Andes más noroccidentales de Suramérica. Geológicamente, la western region of the Western Range in Antioquia and Chocó región noroccidental de la Cordillera Occidental en Antioquia includes the fault resulting from the connection between the y Chocó contiene la falla resultante de la unión entre el Istmo Isthmus of Panamá and South America. The Occidental and de Panamá y Sudamérica. Las cordilleras Occidental y Central Central cordilleras in Colombia are characterized by a num- de Colombia se caracterizan por presentar una cantidad impor- ber of reliefs, valleys and water basins, containing historical tante de relieves, valles y cuencas hidrográficas compuestas por biological refuges and endemisms. -
Akes an Ant an Ant? Are Insects, and Insects Are Arth Ropods: Invertebrates (Animals With
~ . r. workers will begin to produce eggs if the queen dies. Because ~ eggs are unfertilized, they usually develop into males (see the discus : ~ iaplodiploidy and the evolution of eusociality later in this chapter). =- cases, however, workers can produce new queens either from un ze eggs (parthenogenetically) or after mating with a male ant. -;c. ant colony will continue to grow in size and add workers, but at -: :;oint it becomes mature and will begin sexual reproduction by pro· . ~ -irgin queens and males. Many specie s produce males and repro 0 _ " females just before the nuptial flight . Others produce males and ---: : ._ tive fem ales that stay in the nest for a long time before the nuptial :- ~. Our largest carpenter ant, Camponotus herculeanus, produces males _ . -:= 'n queens in late summer. They are groomed and fed by workers :;' 0 it the fall and winter before they emerge from the colonies for their ;;. ights in the spring. Fin ally, some species, including Monomoriurn : .:5 and Myrmica rubra, have large colonies with multiple que ens that .~ ..ew colonies asexually by fragmenting the original colony. However, _ --' e polygynous (literally, many queens) and polydomous (literally, uses, referring to their many nests) ants eventually go through a -">O=- r' sexual reproduction in which males and new queens are produced. ~ :- . ant colony thus functions as a highly social, organ ized "super _ _ " 1." The queens and mo st workers are safely hidden below ground : : ~ - ed within the interstices of rotting wood. But for the ant workers ~ '_i S ' go out and forage for food for the colony,'life above ground is - =- . -
Hymenoptera: Formicidae)
ASIAN MYRMECOLOGY Volume 8, 17 – 48, 2016 ISSN 1985-1944 © Weeyawat Jaitrong, Benoit Guénard, Evan P. Economo, DOI: 10.20362/am.008019 Nopparat Buddhakala and Seiki Yamane A checklist of known ant species of Laos (Hymenoptera: Formicidae) Weeyawat Jaitrong1, Benoit Guénard2, Evan P. Economo3, Nopparat Buddhakala4 and Seiki Yamane5* 1 Thailand Natural History Museum, National Science Museum, Technopolis, Khlong 5, Khlong Luang, Pathum Thani, 12120 Thailand E-mail: [email protected] 2 School of Biological Sciences, The University of Hong Kong, Pok Fu Lam Road, Hong Kong SAR, China 3 Okinawa Institute of Science and Technology Graduate University, 1919-1 Tancha, Onna, Okinawa 904-0495, Japan 4 Biology Divisions, Faculty of Science and Technology, Rajamangala Univer- sity of Technology Tanyaburi, Pathum Thani 12120 Thailand E-mail: [email protected] 5 Kagoshima University Museum, Korimoto 1-21-30, Kagoshima-shi, 890-0065 Japan *Corresponding author’s email: [email protected] ABSTRACT. Laos is one of the most undersampled areas for ant biodiversity. We begin to address this knowledge gap by presenting the first checklist of Laotian ants. The list is based on a literature review and on specimens col- lected from several localities in Laos. In total, 123 species with three additional subspecies in 47 genera belonging to nine subfamilies are listed, including 62 species recorded for the first time in the country. Comparisons with neighboring countries suggest that this list is still very incomplete. The provincial distribu- tion of ants within Laos also show that most species recorded are from Vien- tiane Province, the central part of Laos while the majority of other provinces have received very little, if any, ant sampling. -
Five New Species of Dilobocondyla (Hymenoptera: Formicidae) with a Revised Key to the Known Species
ASIAN MYRMECOLOGY Volume 5, 29–44, 2013 ISSN 1985-1944 © HIMENDER BH ARTI AND RAKES H KUMAR Five new species of Dilobocondyla (Hymenoptera: Formicidae) with a revised key to the known species HIMENDER BH ARTI 1 AND RAKES H KUMAR 2 Department of Zoology & Environmental Sciences, Punjabi University, Patiala, Punjab 147002, India. Corresponding author's emails: 1 [email protected]/[email protected]; 2 [email protected] ABSTRACT. Five new species of Dilobocondyla are described. Dilobocondyla gasteroreticulatus sp. nov. is reported from India based on worker and female castes. Dilobocondyla eguchii sp. nov. and D. propotriangulatus sp. nov. are reported from Vietnam, D. yamanei sp. nov. from Malaysia and D. gaoyureni from China based on worker caste only. Dilobocondyla fouqueti Santschi is redescribed, with reports of male and female castes. A revised key to the genus is also provided. With the addition of these five new species, the genus is now represented by 15 species and two subspecies globally. Keywords: Dilobocondyla, new species, revised key, India, Malaysia, Vietnam, China, Guangdong. INTRODUCTION species. A revised key to all the known species of Dilobocondyla is also provided herewith. Genus Dilobocondyla Santschi, which belongs to tribe Formicoxenini, is a small genus of the MATERIALS AND METHODS subfamily Myrmicinae. It is represented by ten species and two subspecies, from the Oriental The digital images of these species were prepared and Indo-Australian regions (Bolton 2011). All on a Nikon SMZ-1500 stereo zoom microscope species of this genus seem to be rare and most are using Auto-Montage software. Later, images were represented by a single specimen only.