Chapter 23 Patterns and Controls on Historical Channel Change in The
Total Page:16
File Type:pdf, Size:1020Kb
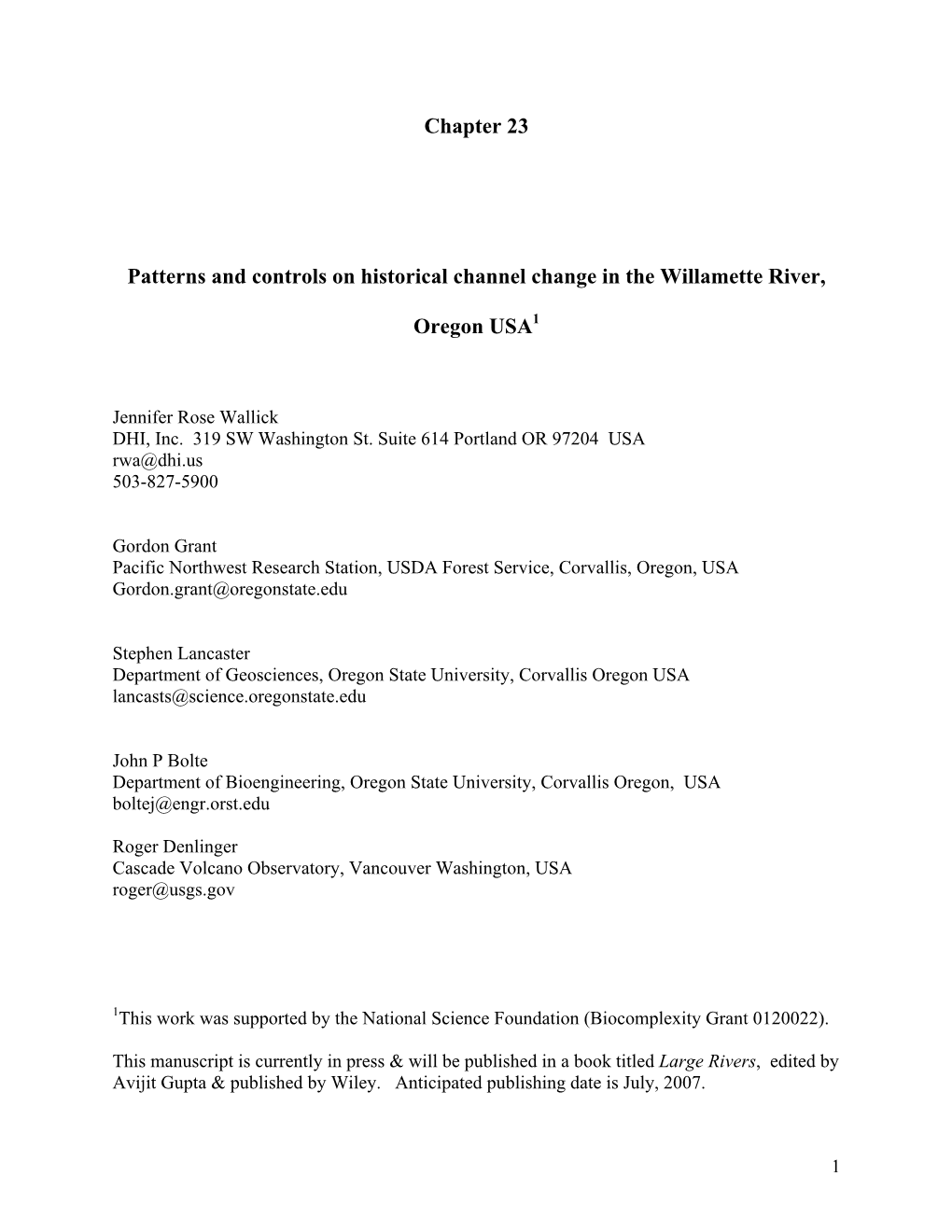
Load more
Recommended publications
-
Land Cover Change Along the Willamette River, Oregon
AN ABSTRACT OF THE THESIS OF Doug R. Oetter for the degree of Doctor of Philosophy in Geography presented on August 16, 2002. Title: Land CoverChange along the Willamette River, Oregon. Abstract approved: A. Jon Kimerling The Willamette River and its floodplain in northwest Oregon have changed dramatically since European settlement. At one time, the river was a vast complex system of braided channels with a broad floodplain forest; it has now been simplified by channelization and dams, and the forest has been removed to support agricultural and urban expansion. This thesis presents three research manuscripts, each of which uses remote sensing and Geographical Information System methods to capture the dynamic nature of land along the Willamette River. In the first article, the river and its floodplain were mapped at four time periods, ranging from pre-settlement to modern day. The paper reports that the river system has been greatly simplified, and the floodplain has lost much of its original forest. An overlay technique was used to identify potential floodplain restoration sites. The second paper details the creation of a landcover map for the Willamette Valley, which shows that the largest portion of the floodplain is now in agriculture, with a significant amount in a built condition. The third manuscript outlines a methodology for detecting land cover change along the river during a twenty-year period that coincides with the implementation of the Willamette River Greenway, a land use designation designed to restrict riparian land conversion. It shows that regressive change is still occuring along the river, although not at thesame rate asoutside the greenway. -
Albany-Calapooia Assessment and Project Implementation Plan
Calapooia River - Albany Assessment and Project Implementation Plan Final Report Site Assessment on the Willamette River. Submitted To: Ms. Tara Davis, Executive Director Calapooia Watershed Council PO Box 844 Brownsville, Oregon 97327 Submitted By: River Design Group, Inc. 311 SW Jefferson Avenue Corvallis, Oregon 97333 April 29, 2011 Calapooia - Albany Assessment and Project Implementation Plan EXECUTIVE SUMMARY The Calapooia Watershed Council and the City of Albany retained River Design Group, Inc. to complete an existing conditions assessment and restoration opportunities prioritization plan for several waterbodies in the vicinity of Albany, Oregon. The assessment reaches include the lower 3 miles of the Calapooia River, the Willamette River and adjacent agricultural areas from RM 114 to RM 122, lower Periwinkle Creek, Thornton Lake, and the Albany oxbow lakes adjacent to the Willamette River. This effort follows the Calapooia River Watershed Assessment (CWC 2004) which provided an evaluation of the drainage at the watershed scale, and the Middle Calapooia Assessment (RDG 2007). This document serves two purposes; one, as an assessment, it presents information on historical and existing conditions based on field data collection, remote sensing, and existing data review. Secondly, the document serves as a river corridor restoration project prioritization plan that highlights restoration actions to address limiting factors and achieve restoration goals. Individual opportunities are identified for each of the target waterbodies. -i- April -
Spatial Patterns of Sediment Transport
SPATIAL PATTERNS OF SEDIMENT TRANSPORT IN THE UPPER WILLAMETTE RIVER, OREGON by TREVOR BROOKS LANGSTON A THESIS Presented to the Department of Geography and the Graduate School of the University of Oregon in partial fulfillment of the requirements for the degree of Master of Science June 2015 THESIS APPROVAL PAGE Student: Trevor Brooks Langston Title: Spatial Patterns of Sediment Transport in the Upper Willamette River, Oregon This thesis has been accepted and approved in partial fulfillment of the requirements for the Master of Science degree in the Department of Geography by: Mark Fonstad Chairperson Patricia McDowell Member and Scott L. Pratt Dean of the Graduate School Original approval signatures are on file with the University of Oregon Graduate School. Degree awarded June 2015 ii © 2015 Trevor Brooks Langston iii THESIS ABSTRACT Trevor Brooks Langston Master of Science Department of Geography June 2015 Title: Spatial Patterns of Sediment Transport in the Upper Willamette River, Oregon The Willamette is a gravel-bed river that drains ~28,800 square kilometers between the Coast Range and Cascade Range in northwestern Oregon before entering the Columbia River near Portland. In the last 150 years, natural and anthropogenic drivers have altered the sediment transport regime, drastically reducing the geomorphic complexity of the river. The purpose of this research is to assess longitudinal trends in sediment transport within the modern flow regime. Sediment transport rates are highly discrete in space, exhibit similar longitudinal patters across flows and increase non- linearly with flow. The highest sediment transport rates are found where the channel is confined due to disconnection of the floodplain and the river runs against high resistance terraces. -
Lower Willamette River Environmental Dredging and Ecosystem Restoration Project
LOWER WILLAMETTE RIVER ENVIRONMENTAL DREDGING AND ECOSYSTEM RESTORATION PROJECT INTEGRATED FEASIBILITY STUDY AND ENVIRONMENTAL ASSESSMENT DRAFT FINAL REPORT March 2015 Prepared by: Tetra Tech, Inc. 1020 SW Taylor St. Suite 530 Portland, OR 97205 This page left blank intentionally EXECUTIVE SUMMARY This Integrated Feasibility Study and Environmental Assessment (FS-EA) evaluates ecosystem restoration actions in the Lower Willamette River, led by the U.S. Army Corps of Engineers (Corps) and the non-Federal sponsor, the City of Portland (City). The study area encompasses the Lower Willamette River Watershed and its tributaries, from its confluence with the Columbia River at River Mile (RM) 0 to Willamette Falls, located at RM 26. The goal of this study is to identify a cost effective ecosystem restoration plan that maximizes habitat benefits while minimizing impacts to environmental, cultural, and socioeconomic resources. This report contains a summary of the feasibility study from plan formulation through selection of a recommended plan, 35% designs and cost estimating, a description of the baseline conditions, and description of impacts that may result from implementation of the recommended plan. This integrated report complies with NEPA requirements. Sections 1500.1(c) and 1508.9(a) (1) of the National Environmental Policy Act of 1969 (as amended) require federal agencies to “provide sufficient evidence and analysis for determining whether to prepare an environmental impact statement or a finding of no significant impact” on actions authorized, funded, or carried out by the federal government to insure such actions adequately address “environmental consequences, and take actions that protect, restore, and enhance the environment." The Willamette River watershed was once an extensive and interconnected system of active channels, open slack waters, emergent wetlands, riparian forests, and adjacent upland forests. -
Raad, Maureen. "Flood Damage and Riparian Forest Restoration
Untitled Document Raad, Maureen. "Flood Damage and Riparian Forest Restoration: Selecting Focal Areas and Setting Restoration Goals on Oregon's Willamette River." Master's Project, University of Oregon, 1999. (Reviewed by Susan Mershon) The title says it all. This Landscape Architecture masters project develops a method for locating areas in the Willamette Basin with high potential for restoration of riparian forests, which are a form of flood control. Carefully chosen areas upstream, which can store water, protect downstream communities from flooding. The large Midwest floods of 1993, and then the 1996 Willamette floods, spurred research into floodplains. Hundred-year floodplains have a 1% chance of flooding each year, and encompass smaller, more frequently Inundated floodplains. Federal policies have altered the "flood flow, floodplain and channel form, and riparian forest cover" to reduce the area of land that gets flooded and make it more suitable for agriculture. This has destroyed many riparian forests. As floodplains are diverse, so are the riparian forests, which can be anywhere within the 100 year floodplain. The riparian forest used to dominate in the Willamette floodplain. Raad creates a trinity typology for the river: geomorphologic features, hydrological processes, and forest types, plus human alterations. She wants to determine which conditions are best for creating riparian forests. She spells out some guiding principles: Complex river channels support many plants and wildlife, and provide more flood storage capacity. Periodic flood inundation and dynamism, channel change, are important to riparian ecosystems. A diverse floodplain makes for diverse ecosystems. Raad assumes all potential restoration sites will lie within the 1996 floodplain. -
Willamette Valley Ecoregion Recovery John Marshall | Geograp
DRAFT FINAL RESEARCH PAPER SPATIAL AUTOCORRELTION PATTERN ANALYSES: Willamette Valley Ecoregion Recovery John Marshall | Geography 517 – Research Paper | June 1, 2017 1 | P a g e John Marshall June 1, 2017 Spring Term 2017 – Geography-517 Geospatial Data Analysis Draft Research Paper Geospatial Characterization and Analyses of Environmental Recovery Actions in the Willamette Valley Ecoregion, Oregon Abstract: Geographers have come to recognize the need to characterize and analyze the state of conservation recovery actions to better understand contemporary ecosystem sustainability. Over twenty mitigation banks and thousands of Federal and State recovery grant projects are now established and ongoing in the Willamette Valley Ecoregion. Both regulatory and nonregulatory recovery programs embrace the notion that solutions should contain elements that support resilience and functionally sustainable systems. The, theory supporting the ranking system used to characterize Willamette Valley ecoregion recovery actions assumes recovery actions are intrinsically important, but more important if they enhance existing natural resources and that their importance is proportional to their size and proximity to one another. A Geographic Information System was selected as a logical technology to create the necessary characterization model and framework for conducting the analyses. Two pattern recognition tools are used to identify statistically significant ecoregion recovery hot spots, cold spots, and spatial outliers. A cursory review and characterization of five hot spot areas identified by the pattern analyses tools was done to help verify whether the model is making appropriate Willamette Valley ecoregion recovery hot spot selections. The model appears to be correctly identifying suitable Willamette Valley ecoregion recovery actions for larger proximate first order hot spots. -
9126 Federal Register / Vol
9126 Federal Register / Vol. 80, No. 33 / Thursday, February 19, 2015 / Rules and Regulations DEPARTMENT OF THE INTERIOR Executive Summary definition of an endangered or This document contains: (1) A final threatened species under the Act. This Fish and Wildlife Service rule to remove the Oregon chub from final rule removes the Oregon chub from the Federal List of Endangered and the Federal List of Endangered and 50 CFR Part 17 Threatened Wildlife, and (2) a notice of Threatened Wildlife. This rule also availability of a final post-delisting removes the currently designated [Docket No. FWS–R1–ES–2014–0002; critical habitat for the Oregon chub FXES11130900000C6–156–FF09E42000] monitoring plan. Species addressed—The Oregon chub throughout its range. Basis for the Regulatory Action— RIN 1018–BA28 (Oregonichthys crameri) is endemic to Under the Act, a species may be the Willamette River drainage of Endangered and Threatened Wildlife determined to be an endangered species western Oregon. Extensive human and Plants; Removing the Oregon or threatened species because of any of activities in the Willamette River Basin Chub From the Federal List of five factors: (A) The present or (e.g., dams, levees, and other human Endangered and Threatened Wildlife threatened destruction, modification, or development within the floodplain) curtailment of its habitat or range; (B) have substantially reduced the amount AGENCY: Fish and Wildlife Service, overutilization for commercial, and suitability of habitat for this Interior. recreational, scientific, or educational species. Improved floodplain ACTION: Final rule. purposes; (C) disease or predation; (D) management and floodplain restoration the inadequacy of existing regulatory SUMMARY: We, the U.S. -
Guidebook for Hydrogeomorphic (HGM)–Based Assessment of Oregon Wetland and Riparian Sites: Statewide Classification and Profiles
Guidebook for Hydrogeomorphic (HGM)–based Assessment of Oregon Wetland and Riparian Sites: Statewide Classification and Profiles Oregon Division of State Lands Guidebook for Hydrogeomorphic (HGM)–based Assessment of Oregon Wetland and Riparian Sites: Statewide Classification and Profiles Prepared for: Oregon Wetland-Riparian Assessment Project Oregon Division of State Lands by: Paul R. Adamus Adamus Resource Assessment, Inc. 6020 NW Burgundy Dr. Corvallis, OR 97330 February 2001 copies available from: Dana Field, Oregon Division of State Lands 775 Summer St. NE Salem, OR 97310 phone: (503) 378-3805 extension 238 email: [email protected] Support for this project was provided by the US Environmental Protection Agency Region 10, and Oregon Division of State Lands This document should be cited as: Adamus, P.R. 2001. Guidebook for Hydrogeomorphic (HGM)–based Assessment of Oregon Wetland and Riparian Sites: Statewide Classification and Profiles. Oregon Division of State Lands, Salem, OR. cover photo courtesy of Steve Shunk - Paradise Birding SUMMARY This guidebook describes a classification system for Oregon wetland and riparian areas based on their hydrogeomorphic (HGM) characteristics: their dominant water sources and setting in the landscape. This represents a regional refinement of a similar national classification. This guidebook provides narrative descriptions (profiles) for each of 14 HGM subclasses that occur in 10 regions of Oregon. The profiles address identification of the subclass, statewide distribution and variability, possible functions, and vulnerability to human-related and natural disturbances. The guidebook provides profiles for 13 natural functions that potentially are valued because they provide services to society. The guidebook documents the occurrence of these functions in wetland/ riparian systems of the Pacific Northwest, describes their potential values and services, and suggests variables and indicators that may predict the relative magnitude of the functions and values. -
Floodplain Restoration at Willamette Mission
R & E Grant Application Project #: 13 Biennium 13-053 Floodplain Restoration at Willamette Mission Project Information R&E Project $47,717.00 Request: Match Funding: $585,589.27 Total Project: $633,306.27 Start Date: 4/26/2014 End Date: 6/30/2015 Project Email: [email protected] Project 13 Biennium Biennium: Organization: Willamette Riverkeeper (Tax ID #: 931212629) Fiscal Officer Name: Marci Krass Address: 1515 SE Water Ave. #102 Portland, OR 97214 Telephone: 503-223-6418 Telephone 2: 307-690-3820 Fax: 503-228-1960 Email: [email protected] Applicant Information Name: Marci Krass Address: 1515 SE Water Ave. Portland, OR 97214 Telephone: 503-223-6418 Email: [email protected] Past Recommended or Completed Projects This applicant has no previous projects that match criteria. Project Summary This project is part of ODFW’s 25 Year Angling Plan. Activity Type: Habitat Summary: Floodplain forest restoration will occur on 450 acres to address limiting factors for Chinook, coho, and steelhead at Willamette Mission State Park, a Conservation Opportunity Area, Willamette anchor habitat, and popular fishing location. Invasive Project #: 13-053 Last Modified/Revised: 12/16/2013 12:31:08 PM Page 1 of 20 Floodplain Restoration at Willamette Mission weeds are preventing forest regeneration in meadows and degrading mature floodplain forest. The project will reestablish native vegetation that will develop into mature forest in the active floodplain, to help meet the habitat complexity, habitat connectivity, food, and water quality needs of native fish. Park users, local youth, and adults will participate in environmental education and on-site stewardship activities as part of the project. -
Willamette River Floodplain Restoration Study Draft Integrated Feasibility Report/ Environmental Assessment
WILLAMETTE RIVER FLOODPLAIN RESTORATION STUDY DRAFT INTEGRATED FEASIBILITY REPORT/ ENVIRONMENTAL ASSESSMENT Lower Coast and Middle Fork Willamette River Subbasins March 2013 Prepared by: U.S. Army Corps of Engineers Tetra Tech, Inc. Portland District Portland, OR Willamette River Floodplain Restoration Study Draft Report This page was intentionally left blank to facilitate double-sided copying. Willamette River Floodplain Restoration Study Draft Report EXECUTIVE SUMMARY This feasibility report presents the results of a U.S. Army Corps of Engineers (Corps) feasibility study undertaken to identify and evaluate alternatives for restoring natural floodplain functions in the lower Coast and Middle Forks of the Willamette River. This report provides documentation of the plan formulation process to select a recommended restoration plan, along with environmental, engineering, and cost details of the recommended restoration plan, which will allow final design and construction to proceed following approval of this report. The study area is the Willamette River Basin of western Oregon. The Willamette River is a major tributary of the Columbia River and is the tenth largest river in the United States, based on average annual flow. This study is being conducted in phases due to the large size and complexity of the Willamette River Basin. Phase 1 of the study involved the development of a framework level plan for the entire Willamette Basin – the Willamette Subbasin Plan (WRI 2004); which documented conditions in the basin and strategies to recover fish and wildlife species as part of the Columbia River Basin Fish and Wildlife Program. Phase 2, the subject of this report, is a feasibility study of floodplain restoration opportunities in the lower Coast and Middle Forks of the Willamette River. -
Wetgrass Prairie Recovery Using the Assistance of an Ecosystem-Wide Conservation and Mitigation Banking Program
SOCIO-ECOLOGICAL CHARACTERIZATION AND MAPPING OF WILLAMETTE VALLEY, OREGON WET PRAIRIE RECOVERY Wetgrass Prairie Recovery Using the Assistance of an Ecosystem-wide Conservation and Mitigation Banking Program University of Washington Geography 560 – Principles of Mapping Winter Quarter 2017 John L. Marshall RECOVERING HISTORICALLY LOST WET PRAIRIE Close to 99% of the pre-European occupation wet grass prairie in the Willamette Valley, Oregon has been converted to pasture and other agricultural uses or urban development. Displacement of aboriginal peoples and their cultural practices of prairie burning to drive game for hunting and maintaining native prairie food plants, such as camas, has led to an accelerated colonization by woody species and nonnative invasive weeds. Over a period of about 15 to 20-years, a mitigation and conservation banking program (ORS 196.668 – 196.622) has been steadily growing around the state of Oregon and 22 of those mitigation banks are established in the Willamette Valley Ecoregion. Many of these mitigation banks are targeting the recovery of Willamette Valley wet grass prairie as part of their over-arching management and long-term protection strategy. A SOCIO-ECOLOGICAL FRAMEWORK FOR WILLAMETTE Human Systems/ Social Ecological Economic Scale VALLEY, OREGON WETGRASS Ecoregion: Native American Extreme Habitat Subsistence to (Willamette Valley, Subsistence/Culture Loss Farm Based PRAIRIE RECOVERY Oregon) to European and Loss of Species to Industrial to Domain Post-Industrial A socio-ecological characterization and mapping framework is used as the Land Parcel Alternative Lifestyle Land Restoration Natural Resource (Muddy Creek Wetland Regulation Adverse Habitat Recovery Based Economy operational construct for meeting the Mitigation Bank) to Regulation Species Recovery project objective. -
Willamette Valley Conservation Study Strategic Habitat Conservation in Oregon’S Willamette Valley
U.S. Fish & Wildlife Service Willamette Valley Conservation Study Strategic Habitat Conservation in Oregon’s Willamette Valley Willamette Valley Conservation Study Strategic Habitat Conservation in Oregon’s Willamette Valley March 2017 Prepared by the: U.S. Department of the Interior Fish and Wildlife Service 911 NE 11th Avenue Portland, Oregon 97232 Recommended citation: U.S. Fish and Wildlife Service. 2017. Willamette Valley Conservation Study. Pacific Region, Portland, Oregon. 148 pp. Willamette Valley Conservation Study Executive Summary Oregon’s Willamette Valley, renowned for the high quality of life it affords its residents, is losing part of its natural heritage. Habitat loss and fragmentation coupled with the loss of disturbance regimes that rejuvenated and maintained native habitats has resulted in population declines of many of the valley’s sensitive wildlife and plants. Several Willamette Valley species are currently listed under the State or Federal endangered species acts. There is a long history of partners working together to address these issues, through land-based and educational conservation actions across the valley. To determine where and how the U.S. Fish and Wildlife Service could complement these actions, we worked with the Oregon Department of Fish and Wildlife, and other governmental and non-governmental organizations engaged in conservation efforts in the Willamette Valley to complete a study of land-based conservation needs and opportunities in the valley. For the purposes of this study, land-based conservation includes actions to identify, preserve, restore, and manage terrestrial areas primarily for their wildlife habitat values while promoting nature-based recreational and educational activities where appropriate. The study concludes that the amount and distribution of lands currently managed for sensitive, native wildlife species is inadequate for depressed populations to recover to a healthy and viable condition across their range in the valley.