Near-Earth Asteroid Resources: Review and Analogue Study of Highly Siderophile Elements in Main-Group Pallasites
Total Page:16
File Type:pdf, Size:1020Kb
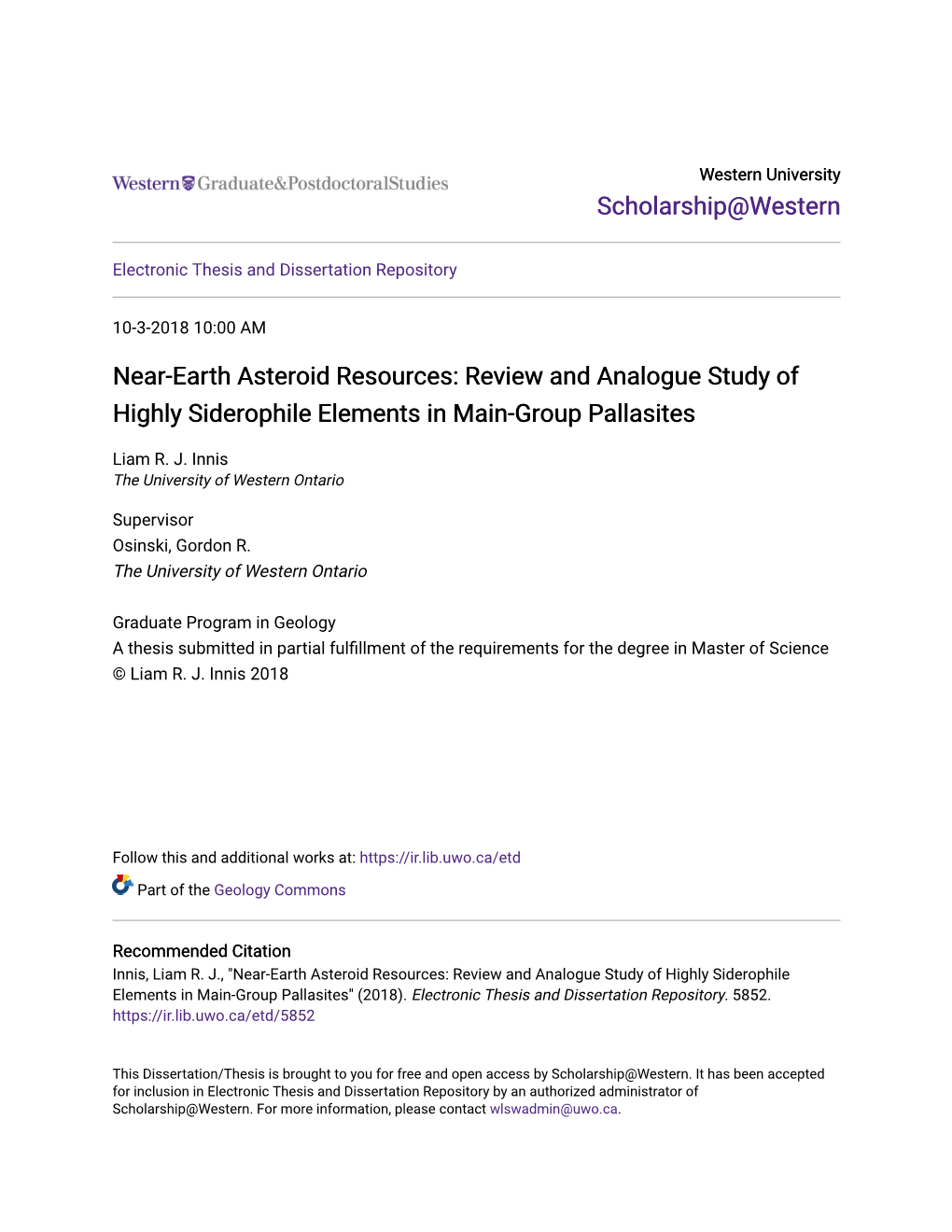
Load more
Recommended publications
-
Space Weathering of Itokawa Particles: Implications for Regolith Evolution
46th Lunar and Planetary Science Conference (2015) 2351.pdf SPACE WEATHERING OF ITOKAWA PARTICLES: IMPLICATIONS FOR REGOLITH EVOLUTION. Eve L. Berger1 and Lindsay P. Keller2, 1Geocontrol Systems – Jacobs JETS contract – NASA Johnson Space Center, Houston TX 77058, [email protected], 2NASA Johnson Space Center Introduction: Space weathering processes such as tion rate of 4.1 ±1.2 × 104 tracks/cm2/year at 1AU [6] solar wind irradiation and micrometeorite impacts are are: ~80,000 years for 0211, ~70,000 years for 0192, known to alter the the properties of regolith materials and ~24,000 years for 0125. exposed on airless bodies [1]. The rates of space Discussion: Cosmic-ray exposure: Measurements weathering processes however, are poorly constrained of cosmic-ray exposure (CRE) ages of Itokawa parti- for asteroid regoliths, with recent estimates ranging cles [6-8] reveal that these grains are relatively young, over many orders of magnitude [e.g., 2, 3]. The return of surface samples by JAXA’s Hayabusa mission to ≤1−1.5 Ma, when compared to the distribution of ex- asteroid 25143 Itokawa, and their laboratory analysis posure ages for LL chondrite meteorites (8−50Ma) [9]. provides “ground truth” to anchor the timescales for The CRE ages for Itokawa grains are consistent with a space weathering processes on airless bodies. Here, we stable regolith at meter-depths for ~106 years. use the effects of solar wind irradiation and the Solar flare particle tracks: Based on the solar flare accumulation of solar flare tracks recorded in Itokawa particle track production rate in olivine at 1AU [6], the grains to constrain the rates of space weathering and Itokawa grains recorded solar flare tracks over time- yield information about regolith dynamics on these scales of <105 years. -
Planetary Science Division Status Report
Planetary Science Division Status Report Jim Green NASA, Planetary Science Division January 26, 2017 Astronomy and Astrophysics Advisory CommiBee Outline • Planetary Science ObjecFves • Missions and Events Overview • Flight Programs: – Discovery – New FronFers – Mars Programs – Outer Planets • Planetary Defense AcFviFes • R&A Overview • Educaon and Outreach AcFviFes • PSD Budget Overview New Horizons exploresPlanetary Science Pluto and the Kuiper Belt Ascertain the content, origin, and evoluFon of the Solar System and the potenFal for life elsewhere! 01/08/2016 As the highest resolution images continue to beam back from New Horizons, the mission is onto exploring Kuiper Belt Objects with the Long Range Reconnaissance Imager (LORRI) camera from unique viewing angles not visible from Earth. New Horizons is also beginning maneuvers to be able to swing close by a Kuiper Belt Object in the next year. Giant IcebergsObjecve 1.5.1 (water blocks) floatingObjecve 1.5.2 in glaciers of Objecve 1.5.3 Objecve 1.5.4 Objecve 1.5.5 hydrogen, mDemonstrate ethane, and other frozenDemonstrate progress gasses on the Demonstrate Sublimation pitsDemonstrate from the surface ofDemonstrate progress Pluto, potentially surface of Pluto.progress in in exploring and progress in showing a geologicallyprogress in improving active surface.in idenFfying and advancing the observing the objects exploring and understanding of the characterizing objects The Newunderstanding of Horizons missionin the Solar System to and the finding locaons origin and evoluFon in the Solar System explorationhow the chemical of Pluto wereunderstand how they voted the where life could of life on Earth to that pose threats to and physical formed and evolve have existed or guide the search for Earth or offer People’sprocesses in the Choice for Breakthrough of thecould exist today life elsewhere resources for human Year forSolar System 2015 by Science Magazine as exploraon operate, interact well as theand evolve top story of 2015 by Discover Magazine. -
Spacewalch Discovery of Near-Earth Asteroids Tom Gehrele Lunar End
N9 Spacewalch Discovery of Near-Earth Asteroids Tom Gehrele Lunar end Planetary Laboratory The University of Arizona Our overall scientific goal is to survey the solar system to completion -- that is, to find the various populations and to study their statistics, interrelations, and origins. The practical benefit to SERC is that we are finding Earth-approaching asteroids that are accessible for mining. Our system can detect Earth-approachers In the 1-km size range even when they are far away, and can detect smaller objects when they are moving rapidly past Earth. Until Spacewatch, the size range of 6 - 300 meters in diameter for the near-Earth asteroids was unexplored. This important region represents the transition between the meteorites and the larger observed near-Earth asteroids (Rabinowitz 1992). One of our Spacewatch discoveries, 1991 VG, may be representative of a new orbital class of object. If it is really a natural object, and not man-made, its orbital parameters are closer to those of the Earth than we have seen before; its delta V is the lowest of all objects known thus far (J. S. Lewis, personal communication 1992). We may expect new discoveries as we continue our surveying, with fine-tuning of the techniques. III-12 Introduction The data accumulated in the following tables are the result of continuing observation conducted as a part of the Spacewatch program. T. Gehrels is the Principal Investigator and also one of the three observers, with J.V. Scotti and D.L Rabinowitz, each observing six nights per month. R.S. McMillan has been Co-Principal Investigator of our CCD-scanning since its inception; he coordinates optical, mechanical, and electronic upgrades. -
Tubular Symplectic Inclusions in Olivine from the Fukang Pallasite
Meteoritics & Planetary Science 45, Nr 5, 899–910 (2010) doi: 10.1111/j.1945-5100.2010.01054.x Tubular symplectic inclusions in olivine from the Fukang pallasite Michael R. STEVENS1, David R. BELL1,2, and Peter R. BUSECK1,2* 1School of Earth and Space Exploration, Arizona State University, Tempe, Arizona 85287, USA 2Department of Chemistry and Biochemistry, Arizona State University, Tempe, Arizona 85287, USA *Corresponding author. E-mail: [email protected] (Received 11 June 2009; revision accepted 27 March 2010) Abstract–Olivine from the Fukang meteorite, like that from many other pallasites, contains distinctive arrays of parallel, straight, tubular inclusions. They differ in their extension and linearity from those in terrestrial olivines. They comprise approximately 1% of the total volume. Most have lens-shaped cross-sections, but some are rounded. The major axis of the lens-shaped inclusions is rigorously oriented along olivine [001], and the rounded ones lie along olivine [010] and a few along [100]. The linear nature and orientations of the inclusions suggest that they nucleated on screw dislocations, perhaps formed through shock triggering. High-resolution transmission electron microscopy (TEM) and energy-dispersive x-ray spectroscopy show that the inclusions consist of symplectic intergrowths of chromite, diopside, and silica that appear to have formed by exsolution from the host olivine. The symplectites consist of chromite lamellae with approximately 35-nm spacings that grew outward from a central plane, with interstitial diopside and silica. Contrast modulations having an average spacing of 4.4 nm occur within the chromite lamellae. Using a reaction- front model, we estimate that exsolution occurred over a period of 30 to 100 min, suggesting rapid cooling at high temperature. -
Rosetta Craft Makes Historic Comet Rendezvous European Space Agency's Comet-Chasing Mission Arrives After 10-Year Journey
NATURE | NEWS Rosetta craft makes historic comet rendezvous European Space Agency's comet-chasing mission arrives after 10-year journey. Elizabeth Gibney 06 August 2014 ESA/Rosetta/MPS for OSIRIS Team MPS/UPD/LAM/IAA/SSO/INTA/UPM/DASP/IDA Comet 67P/Churyumov–Gerasimenko, as seen by Rosetta from a distance of 285 kilometres. No one can deny that it was an epic trip. The European Space Agency's comet-chasing Rosetta spacecraft has arrived at its quarry, after launching more than a decade ago and travelling 6.4 billion kilometres through the Solar System. That makes it the first spacecraft to rendezvous with a comet, and takes the mission a step closer to its next, more ambitious goal of making the first ever soft landing on a comet. Speaking from mission control in Darmstadt, Germany, Matt Taylor, Rosetta project scientist for the European Space Agency (ESA), called the space mission “the sexiest there’s ever been”. Rosetta is now within 100 kilometres of its target, comet 67P/Churyumov–Gerasimenko (or 67P for short), which in July was discovered to be shaped like a rubber duck. After a six-minute thruster burn, at 11:29 a.m. local time on 6 August, ESA scientists confirmed that Rosetta had moved into the same orbit around the Sun as the comet. Rosetta is now moving at a walking pace relative to the motion of 67P — though both are hurtling through space at 15 kilometres per second. Unlike NASA’s Deep Impact and Stardust craft, and ESA’s Giotto mission, which flew by their target comets at high speed, Rosetta will now stay with the comet, taking a ring-side seat as 67P approaches the Sun, and eventually swings around it in August 2015. -
ESO's VLT Sphere and DAMIT
ESO’s VLT Sphere and DAMIT ESO’s VLT SPHERE (using adaptive optics) and Joseph Durech (DAMIT) have a program to observe asteroids and collect light curve data to develop rotating 3D models with respect to time. Up till now, due to the limitations of modelling software, only convex profiles were produced. The aim is to reconstruct reliable nonconvex models of about 40 asteroids. Below is a list of targets that will be observed by SPHERE, for which detailed nonconvex shapes will be constructed. Special request by Joseph Durech: “If some of these asteroids have in next let's say two years some favourable occultations, it would be nice to combine the occultation chords with AO and light curves to improve the models.” 2 Pallas, 7 Iris, 8 Flora, 10 Hygiea, 11 Parthenope, 13 Egeria, 15 Eunomia, 16 Psyche, 18 Melpomene, 19 Fortuna, 20 Massalia, 22 Kalliope, 24 Themis, 29 Amphitrite, 31 Euphrosyne, 40 Harmonia, 41 Daphne, 51 Nemausa, 52 Europa, 59 Elpis, 65 Cybele, 87 Sylvia, 88 Thisbe, 89 Julia, 96 Aegle, 105 Artemis, 128 Nemesis, 145 Adeona, 187 Lamberta, 211 Isolda, 324 Bamberga, 354 Eleonora, 451 Patientia, 476 Hedwig, 511 Davida, 532 Herculina, 596 Scheila, 704 Interamnia Occultation Event: Asteroid 10 Hygiea – Sun 26th Feb 16h37m UT The magnitude 11 asteroid 10 Hygiea is expected to occult the magnitude 12.5 star 2UCAC 21608371 on Sunday 26th Feb 16h37m UT (= Mon 3:37am). Magnitude drop of 0.24 will require video. DAMIT asteroid model of 10 Hygiea - Astronomy Institute of the Charles University: Josef Ďurech, Vojtěch Sidorin Hygiea is the fourth-largest asteroid (largest is Ceres ~ 945kms) in the Solar System by volume and mass, and it is located in the asteroid belt about 400 million kms away. -
Asteroid Retrieval Mission
Where you can put your asteroid Nathan Strange, Damon Landau, and ARRM team NASA/JPL-CalTech © 2014 California Institute of Technology. Government sponsorship acknowledged. Distant Retrograde Orbits Works for Earth, Moon, Mars, Phobos, Deimos etc… very stable orbits Other Lunar Storage Orbit Options • Lagrange Points – Earth-Moon L1/L2 • Unstable; this instability enables many interesting low-energy transfers but vehicles require active station keeping to stay in vicinity of L1/L2 – Earth-Moon L4/L5 • Some orbits in this region is may be stable, but are difficult for MPCV to reach • Lunar Weakly Captured Orbits – These are the transition from high lunar orbits to Lagrange point orbits – They are a new and less well understood class of orbits that could be long term stable and could be easier for the MPCV to reach than DROs – More study is needed to determine if these are good options • Intermittent Capture – Weakly captured Earth orbit, escapes and is then recaptured a year later • Earth Orbit with Lunar Gravity Assists – Many options with Earth-Moon gravity assist tours Backflip Orbits • A backflip orbit is two flybys half a rev apart • Could be done with the Moon, Earth or Mars. Backflip orbit • Lunar backflips are nice plane because they could be used to “catch and release” asteroids • Earth backflips are nice orbits in which to construct things out of asteroids before sending them on to places like Earth- Earth or Moon orbit plane Mars cyclers 4 Example Mars Cyclers Two-Synodic-Period Cycler Three-Synodic-Period Cycler Possibly Ballistic Chen, et al., “Powered Earth-Mars Cycler with Three Synodic-Period Repeat Time,” Journal of Spacecraft and Rockets, Sept.-Oct. -
OSIRIS-Rex, Returning the Asteroid Sample
OSIRIS-REx, Returning the Asteroid Sample Thomas Ajluni Timothy Linn William Willcockson ASRC Federal Space & Defense Lockheed Martin Space Systems Lockheed Martin Space Systems 7000 Muirkirk Meadows Drive Company, P.O. Box 179 Company, P.O. Box 179 Suite 100, Beltsville, MD 20705 Denver, CO 80201 Denver, CO 80201 301.286.1831 303-977-0659 303-977-5094 [email protected] [email protected] [email protected] David Everett Ronald Mink Joshua Wood NASA Goddard Space Flight NASA Goddard Space Flight Lockheed Martin Space Systems Center, 8800 Greenbelt Road Center, 8800 Greenbelt Road Company, P.O. Box 179 Greenbelt, MD 20771 Greenbelt, MD 20771 Denver, CO 80201 301.286.1596 301.286.3524 303-977-3199 [email protected] [email protected] [email protected] Abstract—This paper addresses the technical aspects of the sample return system for the upcoming Origins, Spectral x attitude control Interpretation, Resource Identification, and Security-Regolith x propulsion Explorer (OSIRIS-REx) asteroid sample return mission. The x power overall mission design and current implementation are presented as an overview to establish a context for the x thermal control technical description of the reentry and landing segment of the x telecommunications mission. x command and data handling x structural support to ensure successful rendezvous The prime objective of the OSIRIS-REx mission is to sample a with Bennu primitive, carbonaceous asteroid and to return that sample to Earth in pristine condition for detailed laboratory analysis. x characterization of Bennu’s properties Targeting the near-Earth asteroid Bennu, the mission launches x delivery of the sampler to the surface, and return of in September 2016 with an Earth reentry date of September the spacecraft to the vicinity of the Earth 24, 2023. -
An Overview of Hayabusa2 Mission and Asteroid 162173 Ryugu
Asteroid Science 2019 (LPI Contrib. No. 2189) 2086.pdf AN OVERVIEW OF HAYABUSA2 MISSION AND ASTEROID 162173 RYUGU. S. Watanabe1,2, M. Hira- bayashi3, N. Hirata4, N. Hirata5, M. Yoshikawa2, S. Tanaka2, S. Sugita6, K. Kitazato4, T. Okada2, N. Namiki7, S. Tachibana6,2, M. Arakawa5, H. Ikeda8, T. Morota6,1, K. Sugiura9,1, H. Kobayashi1, T. Saiki2, Y. Tsuda2, and Haya- busa2 Joint Science Team10, 1Nagoya University, Nagoya 464-8601, Japan ([email protected]), 2Institute of Space and Astronautical Science, JAXA, Japan, 3Auburn University, U.S.A., 4University of Aizu, Japan, 5Kobe University, Japan, 6University of Tokyo, Japan, 7National Astronomical Observatory of Japan, Japan, 8Research and Development Directorate, JAXA, Japan, 9Tokyo Institute of Technology, Japan, 10Hayabusa2 Project Summary: The Hayabusa2 mission reveals the na- Combined with the rotational motion of the asteroid, ture of a carbonaceous asteroid through a combination global surveys of Ryugu were conducted several times of remote-sensing observations, in situ surface meas- from ~20 km above the sub-Earth point (SEP), includ- urements by rovers and a lander, an active impact ex- ing global mapping from ONC-T (Fig. 1) and TIR, and periment, and analyses of samples returned to Earth. scan mapping from NIRS3 and LIDAR. Descent ob- Introduction: Asteroids are fossils of planetesi- servations covering the equatorial zone were performed mals, building blocks of planetary formation. In partic- from 3-7 km altitudes above SEP. Off-SEP observa- ular carbonaceous asteroids (or C-complex asteroids) tions of the polar regions were also conducted. Based are expected to have keys identifying the material mix- on these observations, we constructed two types of the ing in the early Solar System and deciphering the global shape models (using the Structure-from-Motion origin of water and organic materials on Earth [1]. -
Near Earth Asteroid (NEA) Scout
https://ntrs.nasa.gov/search.jsp?R=20160007060 2019-08-08T05:23:13+00:00Z Near Earth Asteroid (NEA) Scout Les Johnson NASA MSFC Advance Concepts Office [email protected] Topics • What is a solar sail? • A brief history of solar sailing • NASA’s Near Earth Asteroid Scout mission How does a solar sail work? Solar sails use photon “pressure” or force on thin, lightweight reflective sheet to produce thrust. 3 Topics • What is a solar sail? • A brief history of solar sailing • NASA’s Near Earth Asteroid Scout mission The Planetary Society’s Cosmos-1 (2005) • 100 kg spacecraft • 8 triangular sail blades deployed from a central hub after launch by the inflating of structural tubes. • Sail blades were each 15 m long • Total surface area of 600 square meters • Launched in 2005 from a Russian Volna Rocket from a Russian Delta III submarine in the Barents Sea: The Planetary Society’s Cosmos-1 (2005) • 100 kg spacecraft • 8 triangular sail blades deployed from a central hub after launch by the inflating of structural tubes. • Sail blades were each 15 m long • Total surface area of 600 square meters • Launched in 2005 from a Russian Volna Rocket from a Russian Delta III submarine in the Barents Sea: Rocket Failed NASA Ground Tested Solar Sails in the Mid-2000’s Two 400 square meter sail were autonomously deployed and tested at Plumbrook NanoSail-D Demonstration Solar Sail Mission Description: • 10 m2 sail • Made from tested ground demonstrator hardware NanoSail-D2 Mission (2010) Interplanetary Kite-craft Accelerated by Radiation of the Sun (IKAROS) -
(M = Ca, Mg, Fe2+), a Structural Base of Ca3mg3(PO4)4 Phosphors
crystals Article Crystal Chemistry of Stanfieldite, Ca7M2Mg9(PO4)12 (M = Ca, Mg, Fe2+), a Structural Base of Ca3Mg3(PO4)4 Phosphors Sergey N. Britvin 1,2,* , Maria G. Krzhizhanovskaya 1, Vladimir N. Bocharov 3 and Edita V. Obolonskaya 4 1 Department of Crystallography, Institute of Earth Sciences, St. Petersburg State University, Universitetskaya Nab. 7/9, 199034 St. Petersburg, Russia; [email protected] 2 Nanomaterials Research Center, Kola Science Center of Russian Academy of Sciences, Fersman Str. 14, 184209 Apatity, Russia 3 Centre for Geo-Environmental Research and Modelling, Saint-Petersburg State University, Ulyanovskaya ul. 1, 198504 St. Petersburg, Russia; [email protected] 4 The Mining Museum, Saint Petersburg Mining University, 2, 21st Line, 199106 St. Petersburg, Russia; [email protected] * Correspondence: [email protected] Received: 1 May 2020; Accepted: 25 May 2020; Published: 1 June 2020 Abstract: Stanfieldite, natural Ca-Mg-phosphate, is a typical constituent of phosphate-phosphide assemblages in pallasite and mesosiderite meteorites. The synthetic analogue of stanfieldite is used as a crystal matrix of luminophores and frequently encountered in phosphate bioceramics. However, the crystal structure of natural stanfieldite has never been reported in detail, and the data available so far relate to its synthetic counterpart. We herein provide the results of a study of stanfieldite from the Brahin meteorite (main group pallasite). The empirical formula of the mineral is Ca8.04Mg9.25Fe0.72Mn0.07P11.97O48. Its crystal structure has been solved and refined to R1 = 0.034. Stanfieldite from Brahin is monoclinic, C2/c, a 22.7973(4), b 9.9833(2), c 17.0522(3) Å, β 99.954(2)◦, 3 V 3822.5(1)Å . -
Space Weathering on Mercury
Advances in Space Research 33 (2004) 2152–2155 www.elsevier.com/locate/asr Space weathering on Mercury S. Sasaki *, E. Kurahashi Department of Earth and Planetary Science, The University of Tokyo, Tokyo 113 0033, Japan Received 16 January 2003; received in revised form 15 April 2003; accepted 16 April 2003 Abstract Space weathering is a process where formation of nanophase iron particles causes darkening of overall reflectance, spectral reddening, and weakening of absorption bands on atmosphereless bodies such as the moon and asteroids. Using pulse laser irra- diation, formation of nanophase iron particles by micrometeorite impact heating is simulated. Although Mercurian surface is poor in iron and rich in anorthite, microscopic process of nanophase iron particle formation can take place on Mercury. On the other hand, growth of nanophase iron particles through Ostwald ripening or repetitive dust impacts would moderate the weathering degree. Future MESSENGER and BepiColombo mission will unveil space weathering on Mercury through multispectral imaging observations. Ó 2003 COSPAR. Published by Elsevier Ltd. All rights reserved. 1. Introduction irradiation should change the optical properties of the uppermost regolith surface of atmosphereless bodies. Space weathering is a proposed process to explain Although Hapke et al. (1975) proposed that formation spectral mismatch between lunar soils and rocks, and of iron particles with sizes from a few to tens nanome- between asteroids (S-type) and ordinary chondrites. ters should be responsible for the optical property Most of lunar surface and asteroidal surface exhibit changes, impact-induced formation of glassy materials darkening of overall reflectance, spectral reddening had been considered as a primary cause for space (darkening of UV–Vis relative to IR), and weakening of weathering.