Quantifying Multi-Scaled Movements of Australian Riverine Fish to Inform Environmental Flow Management and Conservation
Total Page:16
File Type:pdf, Size:1020Kb
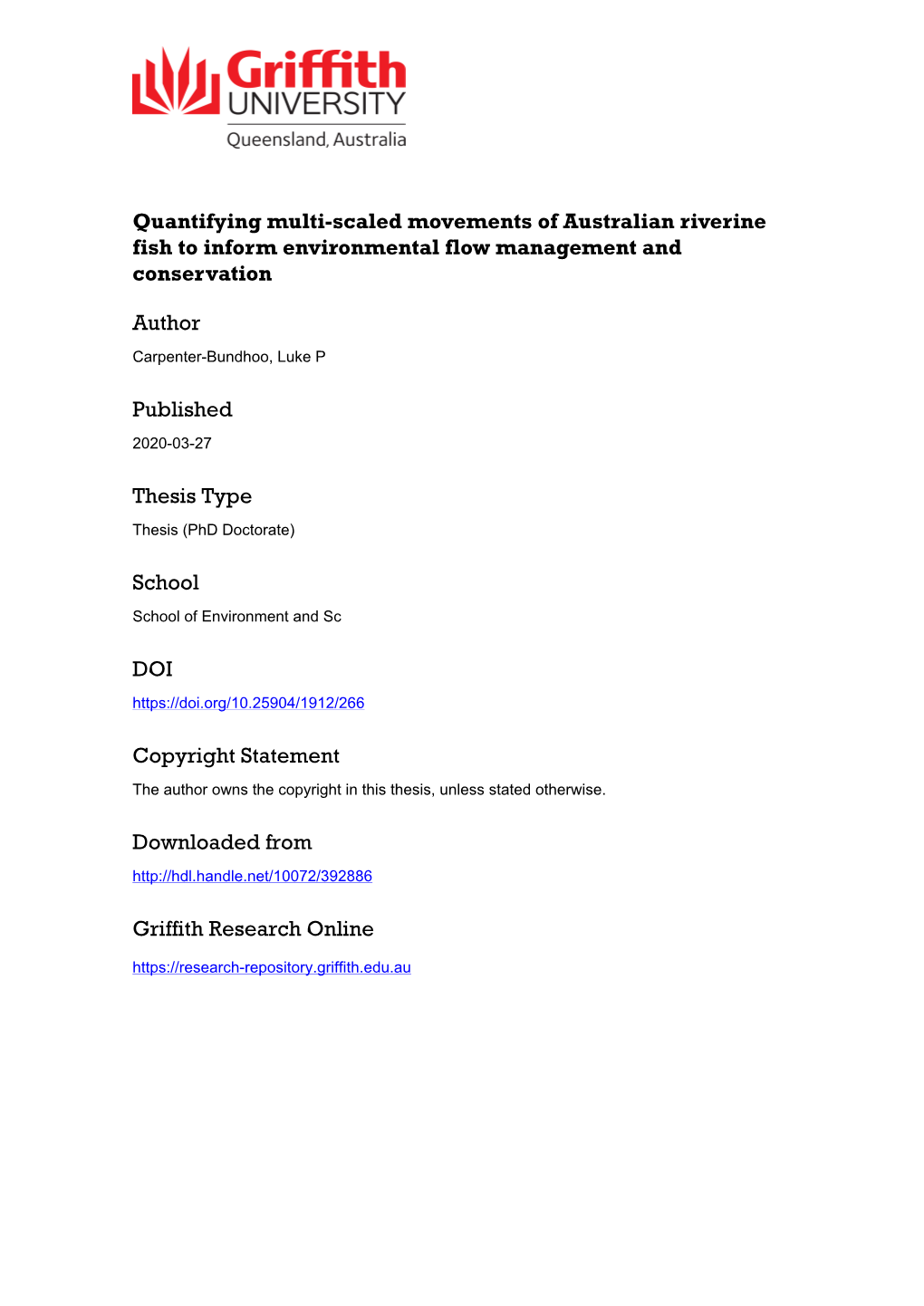
Load more
Recommended publications
-
NSW Strategic Water Information and Monitoring Plan
NSW strategic water information and monitoring plan Water inventory and observation networks in New South Wales IMPORTANT NOTE During the preparation of this report, the following administrative changes occurred in the New South Wales Government: the Department of Water and Energy (DWE) was abolished and the functions relating to the administration of water legislation transferred to the Office of Water within the Department of Environment, Climate Change and Water (DECCW), previously the Department of Environment and Climate Change (DECC). The energy functions of DWE were transferred to the newly created Industry and Investment NSW, previously the Department of Primary Industries (DPI). References throughout this report are to the former agencies. Publisher NSW Office of Water Level 17, 227 Elizabeth Street GPO Box 3889 Sydney NSW 2001 T 02 8281 7777 F 02 8281 7799 [email protected] www.water.nsw.gov.au NSW strategic water information and monitoring plan. Water inventory and observation networks in New South Wales December 2009 ISBN 978 1 921546 94 5 Related publication NSW strategic water information and monitoring plan: Final report Published in December 2009 ISBN 978 1 921546 95 2 Acknowledgements Contributing agencies: NSW Office of Water (the Office), formerly Department of Water and Energy (DWE), Department of Environment, Climate Change and Water (DECCW), formerly Department of Environment and Climate Change (DECC), Industry & Investment NSW, formerly Forests NSW in Department of Primary Industry (DPI), Sydney Catchment Authority This publication may be cited as: Malone D., Torrible L., Hayes J., 2009, NSW strategic water information and monitoring plan: Water inventory and observation networks in New South Wales, NSW Office of Water, Sydney. -
EIS 1177 ABO1 9824 Proposed Granite Quarry
EIS 1177 ABO1 9824 Proposed granite quarry: Gunnalong, Bendemeer NSW: environmental impact statement. kct6 OCO' PROPOSED GRANITE QUARRY GUNNALONG BENDEMEER NSW Environmental Impact Statement NOW DEPAF7ENT OF MNERAL RESOURCES 1 r.J MM November 1995 FORM 4 ENVIRONMENTAL PLANNING AND ASSESSMENT ACT 1979 (SECTION 77(3)(d)) ENVIRONMENTAL IMPACT STATEMENT This statement has been prepared on behalf of Southpac Limited, being the applicant making the development application referred to below. The Statement accompanies the development application made in respect of the development described as follows: Gunnalong Quarry Project The development application relates to the land described as follows: Part Portion Nos. 60, 61, 74, 83, 164 Parish of Bendemeer. The area subject of the application is entirely within Exploration Licence No. 3315 held by Southpac Limited. The contents of this Statement, as required by Clause 34 of the Environmental I Planning and Assessment Regulation 1980, are set out in the accompanying pages. Name, qualifications and address Malcolm John Robinson BSc(Hons) I of person who prepared the Southpac Limited Environmental Impact Statement Level 14 Philips Building 1 15 Blue Street, North Sydney I, Malcolm Robinson of Southpac Limited, hereby certify that I have prepared the contents of this statement in accordance with Clauses 34 and 35 of the Environmental I Planning and Assessment Regulation 1980. Signature /-S ' Iq ~ v-- I CONTENTS I Page 1. SUMMARY 4 I 1.1 Outline of the Proposed Development 4 1.2 The Existing Environment 4 I 1.3 Summary of Environmental Impacts 5 2. INTRODUCTION 7 2.1 General 7 2.2 Corporate Background 7 2.3 Dimension Stone - the Commodity 8 2.3. -
Ken Hill and Darling River Action Group Inc and the Broken Hill Menindee Lakes We Want Action Facebook Group
R. A .G TO THE SOUTH AUSTRALIAN MURRAY DARLING BASIN ROYAL COMMISSION SUBMISSION BY: The Broken Hill and Darling River Action Group Inc and the Broken Hill Menindee Lakes We Want Action Facebook Group. With the permission of the Executive and Members of these Groups. Prepared by: Mark Hutton on behalf of the Broken Hill and Darling River Action Group Inc and the Broken Hill Menindee Lakes We Want Action Facebook Group. Chairman of the Broken Hill and Darling River Action Group and Co Administrator of the Broken Hill Menindee Lakes We Want Action Facebook Group Mark Hutton NSW Date: 20/04/2018 Index The Effect The Cause The New Broken Hill to Wentworth Water Supply Pipeline Environmental health Floodplain Harvesting The current state of the Darling River 2007 state of the Darling Report Water account 2008/2009 – Murray Darling Basin Plan The effect on our communities The effect on our environment The effect on Indigenous Tribes of the Darling Background Our Proposal Climate Change and Irrigation Extractions – Reduced Flow Suggestions for Improvements Conclusion References (Fig 1) The Darling River How the Darling River and Menindee Lakes affect the Plan and South Australia The Effect The flows along the Darling River and into the Menindee Lakes has a marked effect on the amount of water that flows into the Lower Murray and South Australia annually. Alought the percentage may seem small as an average (Approx. 17% per annum) large flows have at times contributed markedly in times when the Lower Murray River had periods of low or no flow. This was especially evident during the Millennium Drought when a large flow was shepherded through to the Lower Lakes and Coorong thereby averting what would have been a natural disaster and the possibility of Adelaide running out of water. -
Murray-Darling Basin Authority Regional Fact Sheet for Lower
Gwydir region Overview The Gwydir region covers The Gwydir catchment is within the 5360 km2 – around 2% of the traditional lands of the Gomeroi/ Murray–Darling Basin. Kamilaroi people. The floodplains of the wydirG Copeton Dam, 35 km south-west of region include wetland Inverell, was built in 1973 to supply vegetation supported by natural water for irrigation, stock and channels, semi-permanent domestic requirements. It regulates wetlands and swamps. 93% of catchment inflows. The region is predominantly The area is a popular tourist agricultural with dryland and destination due to its artesian spa irrigated cropping prominent. water from the Great Artesian Basin. Image: Gwydir Wetlands on the Gwydir River/Gingham Watercourse, New South Wales Carnarvon N.P. r e v i r e R iv e R v i o g N re r r e a v i W R o l g n Augathella a L r e v i R d r a W Chesterton Range N.P. Charleville Mitchell Morven Roma Cheepie Miles River Chinchilla amine Cond Condamine k e e r r ve C i R l M e a nn a h lo Dalby c r a Surat a B e n e o B a Wyandra R Tara i v e r QUEENSLAND Brisbane Toowoomba Moonie Thrushton er National e Riv ooni Park M k Beardmore Reservoir Millmerran e r e ve r i R C ir e e St George W n i Allora b Cunnamulla e Bollon N r e Jack Taylor Weir iv R e n n N lo k a e B Warwick e r C Inglewood a l a l l a g n u Coolmunda Reservoir M N acintyre River Goondiwindi 25 Dirranbandi M Stanthorpe 0 50 Currawinya N.P. -
Murray Cod (Maccullochella Peelii Peelii)
Murray Cod (Maccullochella peelii peelii) Qifeng Ye, G. Keith Jones, and Bryan E. Pierce November 2000 Fishery Assessment Report to PIRSA for the Inland Waters Fishery Management Committee South Australian Fisheries Assessment Series 2000/17 Murray cod (Maccullochella peelii peelii) Murray Cod (Maccullochella peelii peelii) Qifeng Ye, G. Keith Jones, and Bryan E. Pierce November 2000 Fishery Assessment Report to PIRSA for the Inland Waters Fishery Management Committee South Australian Fisheries Assessment Series 2000/17 Murray cod (Maccullochella peelii peelii) i TABLE OF CONTENTS LIST OF TABLES.................................................................................................................................II LIST OF FIGURES............................................................................................................................. III ACKNOWLEDGMENTS..................................................................................................................... V 1. EXECUTIVE SUMMARY ................................................................................................................ 1 2. BACKGROUND................................................................................................................................. 5 2.1. FISHERY ......................................................................................................................................... 5 2.1.1. History ................................................................................................................................... -
Government Gazette No 63 of 16 June 2017 Government Notices GOVERNMENT NOTICES
GOVERNMENT GAZETTE – 16 June 2017 Government Gazette of the State of New South Wales Number 63 Friday, 16 June 2017 The New South Wales Government Gazette is the permanent public record of official notices issued by the New South Wales Government. It also contains local council and other notices and private advertisements. The Gazette is compiled by the Parliamentary Counsel’s Office and published on the NSW legislation website (www.legislation.nsw.gov.au) under the authority of the NSW Government. The website contains a permanent archive of past Gazettes. To submit a notice for gazettal – see Gazette Information. By Authority ISSN 2201-7534 Government Printer 2503 NSW Government Gazette No 63 of 16 June 2017 Government Notices GOVERNMENT NOTICES WaterNSW Prices for rural bulk water services from 1 July 2017 Determination Water Charge (Infrastructure) Rules 2010 (Cth) Independent Pricing and Regulatory Tribunal Act 1992 (NSW) Determination June 2017 Water 2504 NSW Government Gazette No 63 of 16 June 2017 Government Notices © Independent Pricing and Regulatory Tribunal of New South Wales 2017 This work is copyright. The Copyright Act 1968 permits fair dealing for study, research, news reporting, criticism and review. Selected passages, tables or diagrams may be reproduced for such purposes provided acknowledgement of the source is included. ISBN 978-1-76049-083-6 Deter 17-01 The Tribunal members for this review are: Dr Peter J Boxall AO, Chair Mr Ed Willett Ms Deborah Cope Independent Pricing and Regulatory Tribunal of New South Wales -
Survey Guidelines for Australia's Threatened Fish
Survey guidelines for Australia’s threatened fish Guidelines for detecting fish listed as threatened under the Environment Protection and Biodiversity Conservation Act 1999 Authorship and acknowledgments This report updates and expands on a report prepared in May 2004 by Australian Museum ichthyologist John Pogonoski and approved by AMBS Senior Project Manager Jayne Tipping. The current (2011) report includes updates to the 2004 report and additional information regarding recently listed species, current knowledge of all the listed species and current survey techniques. This additional information was prepared by Australian Museum ichthyologists Dr Doug Hoese and Sally Reader. Technical assistance was provided by AMBS ecologists Mark Semeniuk and Lisa McCaffrey. AMBS Senior Project Manager Glenn Muir co- ordinated the project team and reviewed the final report. These guidelines could not have been produced without the assistance of a number of experts. Individuals who have shared their knowledge and experience for the purpose of preparing this report are indicated in Appendix A. Disclaimer The views and opinions contained in this document are not necessarily those of the Australian Government. The contents of this document have been compiled using a range of source materials and while reasonable care has been taken in its compilation, the Australian Government does not accept responsibility for the accuracy or completeness of the contents of this document and shall not be liable for any loss or damage that may be occasioned directly or indirectly through the use of or reliance on the contents of the document. © Commonwealth of Australia 2011 This work is copyright. You may download, display, print and reproduce this material in unaltered form only (retaining this notice) for your personal, non-commercial use or use within your organisation. -
REVOLUTIONISING Inland Fish Production and Stocking in Victoria “WE GROW YOUR FISH”
REVOLUTIONISING Inland Fish Production and Stocking in Victoria “WE GROW YOUR FISH” Prepared by the Snobs Creek Advisory Board Introduction The Victorian Fisheries Authority (VFA) has a long and successful history of growing and stocking fish to recover threatened species, build fish populations and create fantastic recreational fishing opportunities in Victoria. Most of our waterways are heavily modified and no longer consistently provide conditions that support natural breeding for fish. Therefore, fish stocking is an essential and powerful tool for maintaining fish populations and good fishing, alongside river restoration activities including habitat rehabilitation and environmental flows. In Victoria over 200 waters are stocked annually with twelve different fish species. These include both native threatened fish such as Murray cod, golden perch, trout cod, Macquarie perch, silver perch, catfish, Australian bass, and estuary perch, and popular introduced recreational fish species such as brown trout, rainbow trout, chinook salmon, brook trout. Current fish stocking in Victoria ¡[ Mixed Mildura ¡[ ¡[ ¡[ Mixed Mildura¡[ Family Fishing Waters ¡[ ¡[ ¡[ Family Fishing Waters ¡[ Estuarine ¡[ Estuarine ¡[ ¡[ Native ¡[ Mixed ¡[ Native MilduraSwan Hill ¡[ Salmonid ¡[ ¡[ ¡[ ¡[Swan HillFamily Fishing Waters ¡[ Salmonid ¡[¡[ ¡[ ¡[Kerang ¡[ ¡[¡[¡[ Estuarine ¡[ ¡[ ¡[¡[ ¡[ ¡[Kerang ¡[ ¡[ [ ¡[ ¡[ ¡[ ¡[ ¡[¡ [¡[Wodonga¡[ ¡[¡[ Native¡[ ¡[ ¡[ ¡[ ¡[¡[ ¡[ ¡[¡[ ¡[ ¡[ ¡[ ¡[ ¡[ ¡[ ¡[ ¡[ ¡[¡[ Wodonga ¡[ ¡[ ¡[ ¡[ ¡[ ¡[[Shepparton ¡[ ¡[ ¡[¡[ ¡[ ¡[ ¡[ ¡[ ¡[ ¡[¡[ -
2219573-REP-Marine Assessment Report AR
Appendix L – Marine Assessment GHD | Report for Hunter Water Corporation - Belmont Drought Response Desalination Plant, 2219573 Hunter Water Corporation Belmont Drought Response Desalination Plant Marine Environment Assessment Amendment Report July 2020 Table of contents 1. Introduction..................................................................................................................................... 1 1.1 Background .......................................................................................................................... 1 1.2 Purpose and structure of this report .................................................................................... 2 2. Project changes ............................................................................................................................. 4 2.1 Overview .............................................................................................................................. 4 2.2 Key features of the amended Project .................................................................................. 4 3. Methodology ................................................................................................................................... 7 3.1 Review of relevant legislation .............................................................................................. 7 3.2 Review of databases and searches ..................................................................................... 7 3.3 Review of previous marine ecology reports ........................................................................ -
SALINITY SENSITIVITY in EARLY LIFE STAGES of an AUSTRALIAN FRESHWATER FISH, MURRAY COD (Maccullochella Peelii Peelii Mitchell 1838)
i SALINITY SENSITIVITY IN EARLY LIFE STAGES OF AN AUSTRALIAN FRESHWATER FISH, MURRAY COD (Maccullochella peelii peelii Mitchell 1838) Piyapong Chotipuntu THESIS SUBMITTED FOR THE DEGREE OF DOCTOR OF PHILOSOPHY OF THE UNIVERSITY OF CANBERRA Submitted August 2003 ii © Piyapong Chotipuntu 2003 Abstract The Murray cod (Maccullochella peelii peelii Mitchell 1838) is Australia’s largest freshwater fish. Once highly abundant in the Murray-Darling river system, populations have drastically declined in recent decades. Many causes for this decline have been proposed, including over-fishing, habitat loss and altered river flow regimes. This study hypothesised that elevated salinities have led to selective mortality in some developmental stages, which have in turn depleted stock recruitment and adult populations. The objectives of this study were to determine the optimal, threshold, upper sublethal and lethal salinities for development of eggs, yolk-sac larvae, fry and fingerlings of M. peelii peelii. Investigation the impact of salinity on fertilisation utilised gametes of trout cod (M. macquariensis, Cuvier 1829) instead of M. peelii peelii. Studies were carried out in a controlled laboratory environment using test media prepared from commercial sea salt. The results showed that the eggs of the trout cod hatched only when fertilised and incubated in freshwater, and only larvae hatched in freshwater survived through the yolk absorption period of 12 days. Yolk utilisation efficiencies were not significantly different among the salinities of 0-0.30 g/L. There was no effect of pre- or post- fertilising processes on the salinity tolerances of yolk-sac larvae. No larvae survived at salinities higher than 0.30 g/L during the yolk utilisation period. -
Aquatic Ecology of the Montagu River Catchment
Aquatic Ecology of the Montagu River Catchment A Report Forming Part of the Requirements for State of Rivers Reporting David Horner Water Assessment and Planning Branch Water Resources Division DPIWE. December, 2003 State of Rivers Aquatic Ecology of the Montagu Catchment Copyright Notice: Material contained in the report provided is subject to Australian copyright law. Other than in accordance with the Copyright Act 1968 of the Commonwealth Parliament, no part of this report may, in any form or by any means, be reproduced, transmitted or used. This report cannot be redistributed for any commercial purpose whatsoever, or distributed to a third party for such purpose, without prior written permission being sought from the Department of Primary Industries, Water and Environment, on behalf of the Crown in Right of the State of Tasmania. Disclaimer: Whilst DPIWE has made every attempt to ensure the accuracy and reliability of the information and data provided, it is the responsibility of the data user to make their own decisions about the accuracy, currency, reliability and correctness of information provided. The Department of Primary Industries, Water and Environment, its employees and agents, and the Crown in the Right of the State of Tasmania do not accept any liability for any damage caused by, or economic loss arising from, reliance on this information. Preferred Citation: DPIWE (2003). State of the River Report for the Montagu River Catchment. Water Assessment and Planning Branch, Department of Primary Industries, Water and Environment, Hobart. Technical Report No. WAP 03/09 ISSN: 1449-5996 The Department of Primary Industries, Water and Environment The Department of Primary Industries, Water and Environment provides leadership in the sustainable management and development of Tasmania’s resources. -
Diversity and Risk Patterns of Freshwater Megafauna: a Global Perspective
Diversity and risk patterns of freshwater megafauna: A global perspective Inaugural-Dissertation to obtain the academic degree Doctor of Philosophy (Ph.D.) in River Science Submitted to the Department of Biology, Chemistry and Pharmacy of Freie Universität Berlin By FENGZHI HE 2019 This thesis work was conducted between October 2015 and April 2019, under the supervision of Dr. Sonja C. Jähnig (Leibniz-Institute of Freshwater Ecology and Inland Fisheries), Jun.-Prof. Dr. Christiane Zarfl (Eberhard Karls Universität Tübingen), Dr. Alex Henshaw (Queen Mary University of London) and Prof. Dr. Klement Tockner (Freie Universität Berlin and Leibniz-Institute of Freshwater Ecology and Inland Fisheries). The work was carried out at Leibniz-Institute of Freshwater Ecology and Inland Fisheries, Germany, Freie Universität Berlin, Germany and Queen Mary University of London, UK. 1st Reviewer: Dr. Sonja C. Jähnig 2nd Reviewer: Prof. Dr. Klement Tockner Date of defense: 27.06. 2019 The SMART Joint Doctorate Programme Research for this thesis was conducted with the support of the Erasmus Mundus Programme, within the framework of the Erasmus Mundus Joint Doctorate (EMJD) SMART (Science for MAnagement of Rivers and their Tidal systems). EMJDs aim to foster cooperation between higher education institutions and academic staff in Europe and third countries with a view to creating centres of excellence and providing a highly skilled 21st century workforce enabled to lead social, cultural and economic developments. All EMJDs involve mandatory mobility between the universities in the consortia and lead to the award of recognised joint, double or multiple degrees. The SMART programme represents a collaboration among the University of Trento, Queen Mary University of London and Freie Universität Berlin.