Influence of the Temperature-Dependent Dielectric
Total Page:16
File Type:pdf, Size:1020Kb
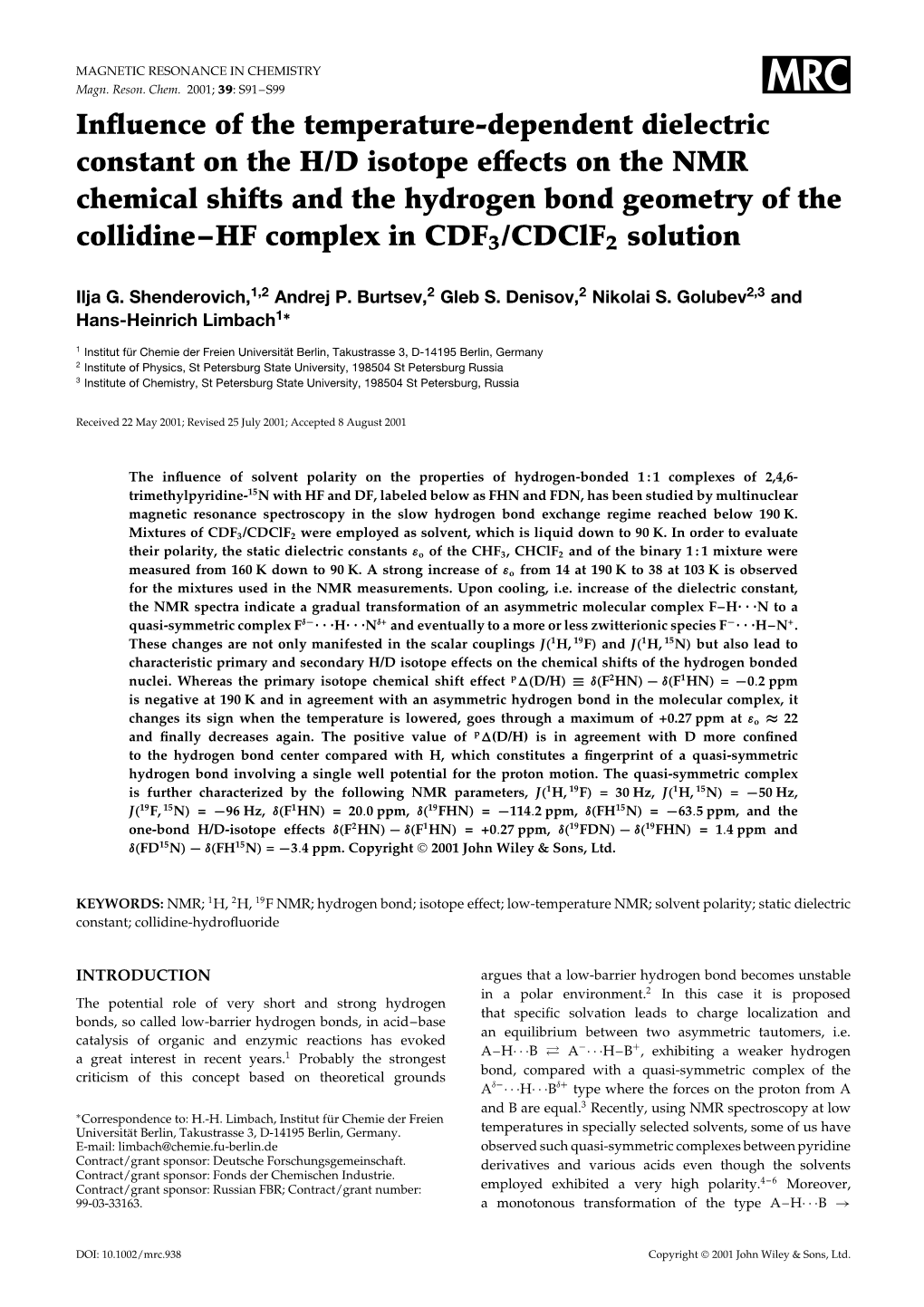
Load more
Recommended publications
-
Sieving Hydrogen Isotopes Through Two Dimensional Crystals
Sieving hydrogen isotopes through two dimensional crystals M. Lozada-Hidalgo1, S. Hu1, O. Marshall1, A. Mishchenko1, A. N. Grigorenko1, R. A. W. Dryfe2, B. Radha1, I. V. Grigorieva1, A. K. Geim1 1School of Physics & Astronomy and 2School of Chemistry, University of Manchester, Manchester M13 9PL, UK One-atom-thick crystals are impermeable to all atoms and molecules but hydrogen ions (thermal protons) penetrate relatively easily through them. We show that monolayers of graphene and boron nitride can be used to separate hydrogen isotopes. Employing electrical measurements and mass spectrometry, we found that deuterons permeate through these crystals much slower than protons, resulting in a large separation factor of ≈10 at room temperature. The isotope effect is attributed to a difference of ≈60 meV between zero-point energies of incident protons and deuterons, which translates into the equivalent difference in the activation barriers posed by two dimensional crystals. In addition to providing insight into the proton transport mechanism, the demonstrated approach offers a competitive and scalable way for hydrogen isotope enrichment. Unlike conventional membranes used for sieving atomic and molecular species, monolayers of graphene and hexagonal boron nitride (hBN) exhibit subatomic selectivity (1-5). They are permeable to thermal protons (5) – and, of course, electrons (6) – but in the absence of structural defects are completely impermeable to larger, atomic species (1-5,7-10). Proton transport through these two-dimensional (2D) crystals was shown to be a thermally activated process, and the found energy barriers E of ≈0.3 and 0.8 eV for monolayers of hBN and graphene, respectively, were attributed to different densities of their electron clouds that have to be pierced by incident protons (5). -
Kinetic Hydrogen/Deuterium Isotope Effects in Multiple Proton Transfer Reactions
Z. Phys. Chem. 218 (2004) 17–49 by Oldenbourg Wissenschaftsverlag, München Kinetic Hydrogen/Deuterium Isotope Effects in Multiple Proton Transfer Reactions By Hans-Heinrich Limbach 1 , ∗, Oliver Klein 1 , Juan Miguel Lopez Del Amo 2, and Jose Elguero 2 1 Institut für Chemie der Freien Universität Berlin, Takustrasse 3, D-14195 Berlin, Germany 2 Instituto de Qu´ımica Medica,´ CSIC, Juan de la Cierva 3, E-28006 Madrid, Spain Dedicated to Prof. Dr. Herbert Zimmermann on the occasion of his 75th birthday (Received May 20, 2003; accepted July 8, 2003) Multiple Proton Transfer / Kinetic Isotope Effects In this paper, a description of multiple kinetic isotope effects (MKIE) of degenerate double, triple and quadruple proton transfer reactions in terms of formal kinetics is developped. Both single and multiple barrier processes are considered, corresponding to so-called “concerted” and “stepwise” reaction pathways. Each step is characterized by a rate constant and the transfer of a given H isotopes or ensemble of H isotopes. The MKIE are expanded in terms of kinetic H/D isotope effects P contributed by single sites in which a proton is transferred in the rate limiting steps. By combination with a modified Bell tunneling model the formalism developed can be used in order to take into account tunneling effects of both concerted and stepwise multiple proton transfers at low temperatures. 1. Introduction Proton transfer constitutes a very complex process, consisting not only of the transfer of a proton but also of diffusion, transport of electrical charges, reori- entation and reorganisation of the solvent molecules, hydrogen bond breaking and forming processes. -
Actual Symmetry of Symmetric Molecular Adducts in the Gas Phase, Solution and in the Solid State
S S symmetry Review Actual Symmetry of Symmetric Molecular Adducts in the Gas Phase, Solution and in the Solid State Ilya G. Shenderovich Institute of Organic Chemistry, University of Regensburg, Universitaetstrasse 31, 93053 Regensburg, Germany; [email protected] Abstract: This review discusses molecular adducts, whose composition allows a symmetric structure. Such adducts are popular model systems, as they are useful for analyzing the effect of structure on the property selected for study since they allow one to reduce the number of parameters. The main objectives of this discussion are to evaluate the influence of the surroundings on the symmetry of these adducts, steric hindrances within the adducts, competition between different noncovalent interactions responsible for stabilizing the adducts, and experimental methods that can be used to study the symmetry at different time scales. This review considers the following central binding units: hydrogen (proton), halogen (anion), metal (cation), water (hydrogen peroxide). Keywords: hydrogen bonding; noncovalent interactions; isotope effect; cooperativity; water; organ- ometallic complexes; NMR; DFT 1. Introduction If something is perfectly symmetric, it can be boring, but it cannot be wrong. If Citation: Shenderovich, I.G. Actual something is asymmetric, it has potential to be questioned. Note, for example, the symmetry Symmetry of Symmetric Molecular of time in physics [1,2]. Symmetry also plays an important role in chemistry. Whether Adducts in the Gas Phase, Solution it is stereochemistry [3], soft matter self-assembly [4,5], solids [6,7], or diffusion [8], the and in the Solid State. Symmetry 2021, dependence of the physical and chemical properties of a molecular system on its symmetry 13, 756. -
Pathways and Mechanisms from Computational Chemistry Calculations
Electronic Supplementary Material (ESI) for Environmental Science: Processes & Impacts. This journal is © The Royal Society of Chemistry 2020 SUPPLEMENTARY INFORMATION Reduction of 1,2,3-Trichloropropane (TCP): Pathways and Mechanisms from Computational Chemistry Calculations Tifany L. Torralba-Sanchez1, Eric J. Bylaska2, Alexandra J. Salter-Blanc3, Douglas E. Meisenheimer1, Molly A. Lyon1, and Paul G. Tratnyek1* 1 OHSU-PSU School of Public Health, Oregon Health & Science University 3181 SW Sam Jackson Park Road, Portland, OR 97239 2 William R. Wiley Environmental Molecular Sciences Laboratory, Pacific Northwest National Laboratory, P.O. Box 999, Richland, WA. 99352 3 Jacobs, 2020 SW 4th Avenue, Suite 300, Portland, OR 97201 *Corresponding author: Email: [email protected], Phone: 503-346-3431 Contents Computational Method Details ............................................................................................. S2 Reactions Modeled, from Figure 2 (Table S1) ...................................................................... S4 Free energies of reaction calculated with hydron (H+) model (Table S2) ............................. S6 + Free energies of reaction calculated with hydronium (H3O ) model (Table S3) ................... S8 Comparison of hydron vs. hydronium modeling (Figure S1) ................................................ S10 Reaction coordinate diagrams with DFT methods (Figures S2-S5) ...................................... S11 Batch Experimental Methods ............................................................................................... -
NAMES for HYDROGEN ATOMS, IONS, and GROUPS, and for REACTIONS INVOLVING THEM (Recommendations 1988)
Pure & Appl. Chem., Vol. 60, No. 7, pp. 11 15-1 11 6, 1988. Printed in Great Britain. @ 1988 IUPAC INTERNATIONAL UNION OF PURE AND APPLIED CHEMISTRY ORGANIC CHEMISTRY DIVISION COMMISSION ON PHYSICAL ORGANIC CHEMISTRY* NAMES FOR HYDROGEN ATOMS, IONS, AND GROUPS, AND FOR REACTIONS INVOLVING THEM (Recommendations 1988) Prepared for publication by J. F. BUNNETT' and R. A. Y. JONES2 'University of California, Santa Cruz, USA *University of East Anglia, Norwich, UK *Membership of the Commission during the period (1983-1987) this report was prepared was as follows: V. Gold? (UK, Chairman: 1983-85); P. Miiller (Switzerland, Chairman: 1985-1987); R. A. Y. Jones (UK, Secretary: 1983-87); T. A. Albright (USA, National Representative); P. Ahlberg (Sweden, National Representative); A. T. Balaban (Romania, National Representative); J. F. Bunnett (USA, Associate); M. P. Doyle (USA, Associate); W. Drenth (Netherlands, National Representative); R. D. Guthrie (USA, Associate); E. A. Halevi (Israel, Titular); J. J. E. Humeres A. (Brazil, National Representative); G. Illuminati? (Italy, Titular); W. P. Jencks (USA, Titular); Jiang Xikui (China, National Representative); P. Laszlo (Belgium, National Representative); J. S. Littler (UK, Associate); D. J. McLennan (New Zealand, National Representative); J. March (USA, Associate); M. Mihailovic (Yugoslavia, National Representative); 0. Nefedov (USSR, Titular); M. Nogradi (Hungary, National Representative); M. Oki (Japan, Titular); J. Penton (Switzerland, Associate); K. Schwetlick (GDR, Titular); J. Toullec (France, Associate); P. van Brandt (Belgium, Associate); Z. Zavada (Czechoslovakia, National Representative); J. Zdysiewicz (Australia, National Representative). t deceased Republication of this report is permitted without the need for formal IUPAC permission on condition that an acknowledgement, with full reference together with IUPAC copyright symbol (0 1988 IUPAC), is printed. -
Es- 2214464- Cluvorres Patented Sept
Sept. 25, 1951 C. E. MORRELL. ET AL 2,569,384 RECOVERY OF OXYGENATED COMPOUNDS FROM HYDROCARBON OILS Filed Dec. 19, l947 ; ; Z O H U C Y - X Charles22g E. 72 ZZ Sr. Verators es- 2214464- Cluvorres Patented Sept. 25, 1951 2,569,384 UNITED STATES PATENT office 2,569,384 RECOVERY OF OXYGENATED COMPOUNDS FROM HYDROCARBON OLS Charles E. Morrell, Westfield, and James E. McAteer, Cranford, N.J., assignors to Standard Oil Development Company, a corporation of Delaware Application December 19, 1947, Serial No. 792,802 1. 1. Claims, (C. 260-450) 2 This invention relates to the recovery of the Oxygen-containing compounds of One to four lower molecular weight neutral oxygen-contain carbon atoms will enter the aqueous phase while ing organic compounds from mixtures thereof the bulk of the compounds containing five car with hydrocarbon oils by a process involving the bon atoms and more per molecule will be found extraction of Such mixtures with dilute aqueous 5 in the oil layer, although it should be borne in carboxylic acid solutions, such as dilute aqueous mind that the separation of materials into their acetic acid, dilute aqueous propionic acid, etc., respective phases is oftentimes not cleancut and Or mixtures of the same. depends to a large extent upon the conditions Warious processes are known to the art in involved and the over-all composition of the Which a mixture of hydrocarbons and Organic O materials in the condensate from the Synthesis Oxygen-containing compounds are produced. reactor. Some of these processes are the low temperature Processes have been developed for the Sepa carbonization of coal, peat and similar materials, ration and recovery of alcohols, ketones, alde the destructive hydrogenation and distillation of hydes, etc. -
Myths About the Proton. the Nature of H+ in Condensed Media. CHRISTOPHER A
Myths about the Proton. The Nature of H+ in Condensed Media. CHRISTOPHER A. REED* Department of Chemistry, University of California, Riverside, California 92521, USA RECEIVED CONSPECTUS Recent research has taught us that most protonated species are decidedly not well represented by a simple + + proton addition. What is the actual nature of the hydrogen ion (the “proton”) when H , HA, H2A etc. are written in formulae, chemical equations and acid catalyzed reactions? In condensed media, H + must be solvated and is nearly always di-coordinate – as illustrated by isolable bisdiethyletherate salts having + H(OEt2)2 cations and weakly coordinating anions. Even carbocations, such as protonated alkenes, have significant C-H---anion hydrogen bonding that gives the active protons two-coordinate character. Hydrogen bonding is everywhere, particularly when acids are involved. In contrast to the normal, asymmetric O-H---O hydrogen bonding found in water, ice and proteins, short, strong, low-barrier (SSLB) H-bonding commonly appears when strong acids are present. Unusually low frequency IR νOHO bands are a good indicator of SSLB H-bonds and curiously, bands associated with group vibrations near H+ in low-barrier H-bonding often disappear from the IR spectrum. + + Writing H3O (the Eigen ion), as often appears in textbooks, might seem more realistic than H for an + + ionized acid in water. However, this, too, is an unrealistic description of H(aq) . The dihydrated H in the + H5O2 cation (the Zundel ion) gets somewhat closer but still fails to rationalize all the experimental and + computational data on H(aq) . Researchers do not understand the broad swath of IR absorption from H(aq) +, known as the “continuous broad absorption” (cba). -
Feature Article
J. Phys. Chem. B 2003, 107, 3351-3366 3351 FEATURE ARTICLE Kinetics of Proton Transport in Water A. A. Kornyshev,*,†,‡ A. M. Kuznetsov,†,§,| E. Spohr,† and J. Ulstrup⊥ Institute for Materials and Processes in Energy Systems (IWV3), Research Center Ju¨lich, D-52425 Ju¨lich, Germany; Department of Chemistry, Faculty of Physical Sciences, Imperial College London, London SW7 2AZ, U.K.; The A. N. Frumkin Institute of Electrochemistry, Russian Academy of Sciences, 117071 Moscow, Russia; Department of Chemistry, The Technical UniVersity of Denmark, DK-2800 Lyngby, Denmark; and Department of Electrochemistry, UniVersity of Ulm, D-89069 Ulm, Germany ReceiVed: April 1, 2002; In Final Form: August 22, 2002 The excess proton mobility in water has attracted scientific attention for more than a century. Detailed theoretical concepts and models are also presently in strong focus in efforts toward understanding this ubiquitous phenomenon. In the present report, we discuss a theoretical framework for rationalizing the excess proton mobility, based on computer simulations, theory of proton transfer (PT) in condensed media, and analysis of classical proton conductivity experiments over broad temperature ranges. The mechanistic options involved + are (i) classical hydrodynamic motion of the hydronium ion (H3O ), (ii) proton transfer from hydronium to + a neighboring water molecule, and (iii) structural diffusion of the Zundel complex (H5O2 ), the processes all controlled by orientational fluctuations or hydrogen bond breaking in neighboring hydration shells. Spontaneous conversion of excess proton states between Zundel and hydrated hydronium states and between hydrated and bare hydronium states are the crucial parts of the scheme. A comparison between experimental data and molecular dynamics (MD) simulations shows that prototropic structural diffusion is determined by comparable contributions of the Zundel and hydrated hydronium states. -
Catalysis by Acetylcholinesterase in Two-Hydronic-Reactive States Integrity of Deuterium Oxide Effects and Hydron Inventories
Biochem. J. (1992) 285, 451-460 (Printed in Great Britain) 451 Catalysis by acetylcholinesterase in two-hydronic-reactive states Integrity of deuterium oxide effects and hydron inventories Erdjan SALIH Department of Chemistry, Brandeis University, Waltham, MA 02254, U.S.A. Low 2H20 effects (1.0-1.5) for the parameter kcat./Km in the hydrolysis of various substrates by acetylcholinesterase (AcChE) is due to normal 2H20 effects (1.8-2.8) for the parameter kcat and 2H20 effects of 1.0-2.5 for the parameter Km, The analyses and interpretations of 2H20 effects in the literature utilizing the parameter kcat/Km, which led to the proposal of 'isotope insensitivity' of the catalytic steps and the hypothesis of a rate-limiting substrate-induced-fit conformational change, are incorrect. Since kcat is the only parameter that can represent the hydron-transfer step solely, the 2H20 effect can most appropriately be evaluated by using this parameter. Calculations and comparison of acylation (k+2) and deacylation (k+3) rate constants show that acylation is rate-determining for most substrates and the improved binding -0.84 to -2.09 kJ/mol (-0.2 to -0.5 kcal/mol) in 2H20 obscures the normal 2H20 effect on kcat when the ratio kcat./Km is utilized. Consistent with this, measurements of the inhibition constant (KI(com.)) for a reversible inhibitor, phenyltrimethylammonium, lead to Kcm) - 39 + 3 tM and K:H2O = 24.5 + 3.5 tM, an 2H20 effect of 1.59 + 0.26. pH-dependence of kca,t in 2H20 is subject to variability of the pK(app.) values, as evaluated in terms of the two-hydronic- reactive states (EH and EH2) of AcChE, and is due to an uneven decrease in 2H20 of the kinetic parameters k'at for the EH2 state relative to kcat for the EH state, thus leading to variable shifts in pK(app,.) values of between 0.5 and 1.2 pH units for this parameter. -
Voltachem in Action 2019
Accelerating industrial electrification VoltaChem in Action 2019 Celebrating our 5th anniversary Join our Shared Innovation Program www.voltachem.com Table of contents Part I The Voltachem Program Page 4 till page 12 Part II Example projects 2019 Page 13 till page 37 Contact information Page 36 till 38 VoltaChem introduction – page 2 VoltaChem introduction – page 3 VoltaChem in short Our mission and strategy • Public-Private Shared Innovation Our mission Our strategy Program of 8M/year initiated in 2015 It is VoltaChem’s mission to support the chemical, electricity, VoltaChem pushes technological developments from TRL 3-5 and equipment supply sectors to find collaborative business (research & innovation) towards TRL 5-7 (piloting and by TNO, ECN, and Topsector Chemistry opportunities and address their challenges together by linking demonstration) in a collaborative program. We do this by (now Holland Chemistry). flexible electricity supply to energy demand in the chemical advancing knowledge through collaboration with top-players sector and by using electricity directly in chemical processing, in the field and by further developing this knowledge into CO2 FERTILIERS employing novel technologies, and business models. pilots and demonstrators together with industry and regional clusters. POWER • Accelerate innovation and implementation WIND -2- CHEMICALS of industrial electrification for reducing CO2 footprint in chemicals production. CHEMICAL POWER POWER BILDING BLOCKS -2- -2- HEAT HYDROGEN • Initiate and facilitate collaborative OUR FOCUS ENERGY BIOMASS STORAGE development of technology and associated business models. TRANSPORT FELS P • Addresses both the indirect and direct use of electricity within the chemical industry, involving stakeholders from chemicals, DEMONSTRATION COMMERCIAL IDEA LAB PILOT energy & equipment supply. -
Comprehensive Review of Current Endodontic Sealers
University of New England DUNE: DigitalUNE Dental Medicine Faculty Publications Dental Medicine Faculty Works 2020 Comprehensive Review Of Current Endodontic Sealers Takashi Komabayashi Clinical Professor, University of New England College of Dental Medicine, [email protected] David Colmenar University of New England, [email protected] Nicholas Cvach University of New England, [email protected] Aparna Bhat University of New England, [email protected] Carolyn Primus Augusta University See next page for additional authors Follow this and additional works at: https://dune.une.edu/cdm_facpubs Part of the Dental Materials Commons, and the Endodontics and Endodontology Commons Recommended Citation Komabayashi, Takashi; Colmenar, David; Cvach, Nicholas; Bhat, Aparna; Primus, Carolyn; and Imai, Yohji, "Comprehensive Review Of Current Endodontic Sealers" (2020). Dental Medicine Faculty Publications. 14. https://dune.une.edu/cdm_facpubs/14 This Article is brought to you for free and open access by the Dental Medicine Faculty Works at DUNE: DigitalUNE. It has been accepted for inclusion in Dental Medicine Faculty Publications by an authorized administrator of DUNE: DigitalUNE. For more information, please contact [email protected]. Authors Takashi Komabayashi, David Colmenar, Nicholas Cvach, Aparna Bhat, Carolyn Primus, and Yohji Imai This article is available at DUNE: DigitalUNE: https://dune.une.edu/cdm_facpubs/14 Dental Materials Journal 2020; 39(5): 703–720 Review Comprehensive review of current endodontic sealers 1 1 1 1 2 3 Takashi KOMABAYASHI , -
Som E K Inetic and Equilibrium Isotope E Ffects in The
Some Kinetic and Equilibrium Isotope Effects in the Isobutylphenone Enol and Enolate Ion System Y. Chiang, A. J. Kresge, and P. A. Walsh Department of Chemistry, University of Toronto, Toronto, Ontario M5S 1A1, Canada Z. Naturforsch. 44a, 406-412 (1989); received November 18, 1988 This paper is dedicated to Professor Jacob Bigeleisen on the occasion of his 70th birthday The following kinetic isotope effects were determined for acid-catalyzed ketonization of isobutyro- phenone enol and enolate ion through rate-determining hydron transfer from catalyst to substrate: enol, kH/kD = 3.30±0.07 (hydronium ion catalysis), /cH//cD = 4.0 + 2.8 (acetic acid catalysis); enolate ion, kH/kD= 1.00 + 0.21 (hydronium ion catalysis), kH/kD = 3A \ +0.20 (acetic acid catalysis), kH /kD = 7.48 + 0.23 (water catalysis). The magnitude of these isotope effects, when assessed in terms of the free energies of reaction for the processes in which they occur, are consistent with Melander-West- heimer-Bigeleisen theory. An equilibrium isotope effect of KH/KD = 5.88 + 0.32 was also determined for the ionization of isobutyrophenone enol as an oxygen acid. There has been a renaissance of interest in the Experimental Section chemistry of simple enols lately, fueled by the inven tion of methods for generating these unstable species Materials. Isobutyrophenone enol was generated in in solution in greater than equilibrium amounts under aqueous solution by hydrolysis of its potassium or conditions where they can be observed directly. This lithium enolate, as described [2 b]. Isobutyrophenone has allowed accurate measurement of rate and equi enol ether, 2, was prepared by acid-catalyzed elimina librium constants for their reactions, and that has led tion of methanol from isobutyrophenone dimethyl to much new enol chemistry [1], In this paper we add ketal, which in turn was made from isobutyrophenone some kinetic and equilibrium isotope effects to this and methanol using a standard method [3].