An Example from the Central Mojave Metamorphic Core Complex
Total Page:16
File Type:pdf, Size:1020Kb
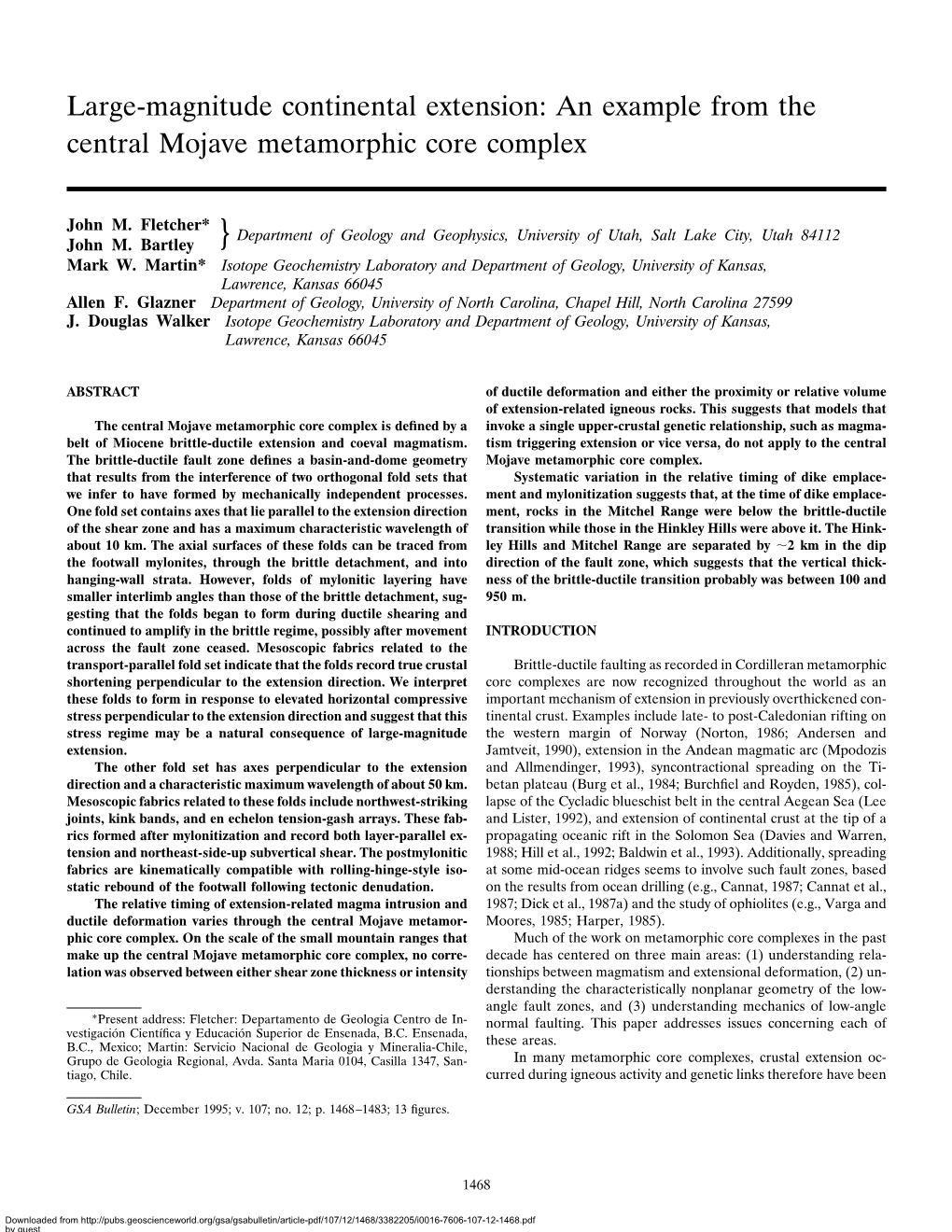
Load more
Recommended publications
-
Pressure-Temperature Evolution of Metapelites Within the Anaconda Metamorphic Core Complex, Southwestern Montana
University of Montana ScholarWorks at University of Montana Graduate Student Theses, Dissertations, & Professional Papers Graduate School 2008 PRESSURE-TEMPERATURE EVOLUTION OF METAPELITES WITHIN THE ANACONDA METAMORPHIC CORE COMPLEX, SOUTHWESTERN MONTANA Erin Haney The University of Montana Follow this and additional works at: https://scholarworks.umt.edu/etd Let us know how access to this document benefits ou.y Recommended Citation Haney, Erin, "PRESSURE-TEMPERATURE EVOLUTION OF METAPELITES WITHIN THE ANACONDA METAMORPHIC CORE COMPLEX, SOUTHWESTERN MONTANA" (2008). Graduate Student Theses, Dissertations, & Professional Papers. 1262. https://scholarworks.umt.edu/etd/1262 This Thesis is brought to you for free and open access by the Graduate School at ScholarWorks at University of Montana. It has been accepted for inclusion in Graduate Student Theses, Dissertations, & Professional Papers by an authorized administrator of ScholarWorks at University of Montana. For more information, please contact [email protected]. PRESSURE-TEMPERATURE EVOLUTION OF METAPELITES WITHIN THE ANACONDA METAMORPHIC CORE COMPLEX, SOUTHWESTERN MONTANA By Erin Marie Haney B.S. Geology, Sonoma State University, Rohnert Park, CA, 2005 Thesis presented in partial fulfillment of the requirements for the degree of Master of Science in Geosciences The University of Montana Missoula, MT Spring 2008 Approved by: Dr. David A. Strobel, Dean Graduate School Dr. Julie Baldwin, Chair Department of Geosciences Dr. James Sears Department of Geosciences Dr. Paul Wilson Department of Geography Haney, Erin, M.S., May 2008 Geosciences Pressure-temperature evolution of metapelites within the Anaconda metamorphic core complex, southwestern Montana Chairperson: Dr. Julie Baldwin This study shows the complete metamorphic evolution of metapelitic footwall rocks in the Anaconda metamorphic core complex, located in southwestern Montana. -
Large-Magnitude Extensional Deformation in the South Mountains Metamorphic Core Complex, Arizona: Evaluation with Paleomagnetism
Large-magnitude extensional deformation in the South Mountains metamorphic core complex, Arizona: Evaluation with paleomagnetism Richard F. Livaccari Department of Earth and Planetary Sciences, University of New Mexico, John W. Geissman } Albuquerque, New Mexico 87131 Stephen J. Reynolds Department of Geology, Arizona State University, Tempe, Arizona 85287 ABSTRACT data from these two sides suggests that the INTRODUCTION back-dipping mylonitic front was synkine- Paleomagnetic data are used to test con- matically tilted about 10 down-to-the- Cordilleran metamorphic core complexes troversial aspects of Cordilleran metamor- southwest. The data support a folded shear (MCCs) and their associated structures (de- phic core complexes, including the original zone hypothesis for origin of the mylonitic tachment faults and mylonites) are the in- dip of extensional structures, origin of the front and the interpretation that footwall ferred products of large-magnitude Cenozo- mylonitic front, and applicability of rolling- rocks possess primary, Miocene-age TRMs ic extension (tens of kilometers of normal hinge models. We obtained paleomagnetic or TCRMs. A second regional fold test in- slip; e.g., Crittenden et al., 1980; Reynolds data (115 sites, 82 accepted for analysis) volved data from sites on both flanks of and Spencer, 1985; Howard and John, 1987; from the weakly deformed interior of a syn- the topographically prominent northeast- Coney, 1987). Aspects of metamorphic core kinematic, footwall intrusive suite and Prot- trending mountain range–scale antiform. complex evolution not fully understood in- erozoic footwall rocks of the South Moun- The negative result from this fold test dem- clude the origin of the mountain range–scale tains metamorphic core complex, central onstrates that this structure formed early in antiforms that strike parallel with the exten- Arizona. -
Metamorphic Core Complexes
Encyclopedia of Marine Geosciences DOI 10.1007/978-94-007-6644-0_104-4 # Springer Science+Business Media Dordrecht 2014 Metamorphic Core Complexes Uwe Ring* Department of Geological Sciences, Stockholm University, Stockholm, Sweden Definition Metamorphic core complexes result from horizontal lithospheric extension and form in low-viscosity lower crust when extension occurs at high rates and deformation within the upper crust becomes localized in detachment faults. They are oval shaped usually updomed structures in which mid-crustal basement rocks of higher metamorphic grade have been tectonically juxtaposed against low-grade upper crustal rocks. Introduction Extension of Earth’s lithosphere is one of the most fundamental processes that shape the face of our planet. Extension and breakup of continental lithosphere is key to understand the evolution of continents, the origin of sedimentary basins, and their hydrocarbon potential, as well as the thermohaline circulation in the oceans and thus global climate. The most spectacular form of extension tectonics is the formation of metamorphic core com- plexes. Metamorphic core complexes mainly develop in continental crust, especially where it has been previously thickened by collisional processes. These processes heated up the thickened crust mainly by radioactive decay thereby weakening it and ultimately causing its failure. In oceans, metamorphic core complexes may form as well near mid-ocean ridges when magma supply is not efficient enough to accommodate extension (e.g., North Atlantic; Tucholke et al., 1998). This entry covers continental core complexes only. What Is a Core Complex? Metamorphic core complexes are usually oval-shaped bodies in map view their long axis is typically some 20–50 km long. -
1 ZEYNEP ONER BARAN Assistant Professor of Geology
SD School of Mines and Technology Department of Geology and Geol. Eng. 501 East Saint Joseph Street 57701 Rapid City, SD (605) 394 5286 [email protected] ZEYNEP ONER BARAN Assistant Professor of Geology EDUCATION Ph.D. Geology: Miami University, Oxford OH (2005 – 2012) B.Sc. Geological Engineering: Istanbul University, Istanbul, Turkey (2000-2004) INTERNSHIP AND FIELD EXPERIENCE Internship: (June 2005-August 2005) MTA (Mineral Research and Exploration Institute, Ankara, Turkey) Field studies: - (May, 2015) Research field trip to Keystone Gold mine district, visiting Mineral Mountain Mine Company for research collaboration - (March, 2015) Research field trip between Keystone, Deadwood, and Sturgis areas: Sampling Tertiary intrusions for structural and petrographic analysis - (November, 2014) Research meeting and geology field trip in Marigold Mine District, Silver Standard Mining Co. - (September, 2013) Structural Geology Field Trip: Understanding extensional structures in Reva Gap, SD - (October 6, 2010) Mid-Congress Field Trip (GSA Tectonic crossroads, Ankara-Turkey): Gerede section of the North Anatolian fault zone - (March 30-April 3, 2009) Field Trip (Global Tectonics course): Tectonics & geomorphology of the Owen Valley, Eastern California shear zone - (June 2008 to August 2008) PhD research project: Systematic sampling of footwall rocks along the Alasehir detachment fault, Alasehir Basin (Western Turkey) - (November, 2008) Field Trip (Structural Geology course): Compressional and extensional structures in the southern Appalachian -
Cordilleran Metamorphic Core Complexes: an Overview
Chapter I CORDILLERAN METAMORPHIC CORE COMPLEXES: AN OVERVIEW by Peter J. Coney INTRODUCTION Cordilleran metamorphic core complexes are a group of usually domal or arch-like, isolated uplifts of anomously deformed, meta-· morphic and plutonic rocks,overlain by a tectonically detached and distended unmetamorphosed cover. The features (see Figure 1-1) are scattered in a sinuous string along the axis of the eastern two-thirds of the North American Cordillera from southern Canada to northwestern Mexico. To date, over 25 of them are known and it is significant that over half of them have been recognized only since 1970. They are, without question, the newest and most con troversial addition to the recognized architecture of the eastern two-thirds of the Cordillera since the discovery, in the early 1960~, of the Tertiary calderas and their associated vast ignim brite sheets. During the past 10 years, considerable debate has centered on them, and they have been termed metamorphic core com plexes (Coney, 1973a, 1976, 1978, 1979; Davis and Coney, 1979; Crittenden et al., 1978). The first hint of the exis tence and potential significance of these complexes was from the early efforts of Peter Misch and his students who began work in the Great Basin in the 1950s. Misch (1960) discovered and emphasized scattered occurrences of a major sub-horizontal dislocation plane or "decollement, as it was called, separating an unmetamorphosed Paleozoic miogeoclinal cover from a usually metamorphosed substratum. He found this repeatedly 25 in many ranges along and west of the Utah-Nevada, border about 200 kms west of the already known low- angle, east-verging Mesozoic thrust faults in central Utah. -
An Example from the Central Mojave Metamorphic Core Complex
Large-magnitude continental extension: An example from the central Mojave metamorphic core complex John M. Fletcher* Department of Geology and Geophysics, University of Utah, Salt Lake City, Utah 84112 John M. Bartley } Mark W. Martin* Isotope Geochemistry Laboratory and Department of Geology, University of Kansas, Lawrence, Kansas 66045 Allen F. Glazner Department of Geology, University of North Carolina, Chapel Hill, North Carolina 27599 J. Douglas Walker Isotope Geochemistry Laboratory and Department of Geology, University of Kansas, Lawrence, Kansas 66045 ABSTRACT of ductile deformation and either the proximity or relative volume of extension-related igneous rocks. This suggests that models that The central Mojave metamorphic core complex is defined by a invoke a single upper-crustal genetic relationship, such as magma- belt of Miocene brittle-ductile extension and coeval magmatism. tism triggering extension or vice versa, do not apply to the central The brittle-ductile fault zone defines a basin-and-dome geometry Mojave metamorphic core complex. that results from the interference of two orthogonal fold sets that Systematic variation in the relative timing of dike emplace- we infer to have formed by mechanically independent processes. ment and mylonitization suggests that, at the time of dike emplace- One fold set contains axes that lie parallel to the extension direction ment, rocks in the Mitchel Range were below the brittle-ductile of the shear zone and has a maximum characteristic wavelength of transition while those in the Hinkley Hills were above it. The Hink- about 10 km. The axial surfaces of these folds can be traced from ley Hills and Mitchel Range are separated by ;2 km in the dip the footwall mylonites, through the brittle detachment, and into direction of the fault zone, which suggests that the vertical thick- hanging-wall strata. -
Late-Stage Exhumation of Metamorphic Core Complexes and Landscape Development During Orogenic Collapse of the North American
LATE-STAGE EXHUMATION OF METAMORPHIC CORE COMPLEXES AND LANDSCAPE DEVELOPMENT DURING OROGENIC COLLAPSE OF THE NORTH AMERICAN CORDILLERA A DISSERTATION SUBMITTED TO THE FACULTY OF GRADUATE SCHOOL OF THE UNIVERSITY OF MINNESOTA BY ERKAN TORAMAN IN PARTIAL FULFILLMENT OF THE REQUIREMENTS FOR THE DEGREE OF DOCTOR OF PHILOSOPHY CHRISTIAN TEYSSIER, DONNA L. WHITNEY, ADVISORS FEBRUARY 2014 © Erkan Toraman, 2014 Acknowledgements First and foremost, I would like to thank my advisors, Christian Teyssier and Donna L. Whitney, for their continuous support throughout the years. Without your guidance, patience, and constant encouragement this work would not be possible. You have provided me freedom to pursue many aspects of this research, even though at times when I wasn’t sure if that particular approach would work. Thank you for long discussions that broadened my understanding of science and academic life in general. I can’t express my gratitude for involving me with many other projects you work on, especially the ones in central Turkey, which allowed me to explore different perspectives on Anatolian tectonics and use new research tools. I hope and look forward to work with you in future projects! Many thanks to members of my written, oral, and final exam committee members Martin O. Saar, Karen Kleinspehn, Joshua Feinberg, and Annia Fayon for taking the time to read my work. I am indebted to Annia Fayon for introducing me to the wonders of fission-track analysis and for long, enlightening discussions on diffusional processes in thermochronology. I thank Martin Saar for his encouragement for modeling of thermochronometric data, even though that work has not made into this thesis but still “in progress”. -
Tectonic Evolution and Paleogeography of the Kirşehir
Tectonic evolution and paleogeography of the Kirşehir Block and the Central Anatolian Ophiolites, Turkey Douwe van Hinsbergen, Marco Maffione, Alexis Plunder, Nuretdin Kaymakcı, Morgan Ganerød, Bart Hendriks, Fernando Corfu, Derya Gürer, Giovanni de Gelder, Kalijn Peters, et al. To cite this version: Douwe van Hinsbergen, Marco Maffione, Alexis Plunder, Nuretdin Kaymakcı, Morgan Ganerød, et al.. Tectonic evolution and paleogeography of the Kirşehir Block and the Central Anatolian Ophiolites, Turkey. Tectonics, American Geophysical Union (AGU), 2016, 35 (4), pp.983-1014. 10.1002/2015TC004018. insu-01470256 HAL Id: insu-01470256 https://hal-insu.archives-ouvertes.fr/insu-01470256 Submitted on 17 Feb 2017 HAL is a multi-disciplinary open access L’archive ouverte pluridisciplinaire HAL, est archive for the deposit and dissemination of sci- destinée au dépôt et à la diffusion de documents entific research documents, whether they are pub- scientifiques de niveau recherche, publiés ou non, lished or not. The documents may come from émanant des établissements d’enseignement et de teaching and research institutions in France or recherche français ou étrangers, des laboratoires abroad, or from public or private research centers. publics ou privés. PUBLICATIONS Tectonics RESEARCH ARTICLE Tectonic evolution and paleogeography of the Kırşehir 10.1002/2015TC004018 Block and the Central Anatolian Ophiolites, Turkey Key Points: Douwe J. J. van Hinsbergen1, Marco Maffione1, Alexis Plunder1,2, Nuretdin Kaymakcı3, • Central Anatolia formed due to 4 4,5 6 1 1,7 interplay of two simultaneous Morgan Ganerød ,BartW.H.Hendriks , Fernando Corfu , Derya Gürer , Giovanni I. N. O. de Gelder , 1 1 8 1,9 1 subduction zones Kalijn Peters , Peter J. -
Continental and Oceanic Core Complexes 1888 2013
Continental and oceanic core complexes 1888 2013 CELEBRATING ADVANCES IN GEOSCIENCE 1,† 1 2 3 Donna L. Whitney , Christian Teyssier , Patrice Rey , and W. Roger Buck Invited Review 1Department of Earth Sciences, University of Minnesota, Minneapolis, Minnesota 55455, USA 2School of Geosciences, University of Sydney, Sydney NSW 2006, Australia 3Lamont-Doherty Earth Observatory, Columbia University, Palisades, New York 10964, USA ABSTRACT rocks ± upper mantle in the footwall of the nor- Core complexes were fi rst recognized in the mal fault(s). The resulting structure is a core continents (e.g., Anderson, 1972; Coney, 1974, Core-complex formation driven by litho- complex, which occurs in both continental and 1980; Crittenden et al., 1980; Lister and Davis, spheric extension is a fi rst-order process of oceanic lithosphere (Figs. 1 and 2). Extension is 1989), and they have been identifi ed in the geo- heat and mass transfer in the Earth. Core- the direct driving force for core-complex devel- logic record from the Precambrian (Holm, 1996) complex structures have been recognized opment, but in continental settings, the far-fi eld to the present (Hill et al., 1992). Core complexes in the continents, at slow- and ultraslow- tectonic regime may be one of convergence, and were later identifi ed at slow- and ultraslow- spreading mid-ocean ridges, and at continen- therefore continental core complexes may occur spreading oceanic divergent zones (e.g., Cannat, tal rifted margins; in each of these settings, in orogenic settings under an overall regime of 1993; Cann et al., 1997; Blackman et al., 1998; extension has driven the exhumation of deep plate convergence. -
Extension Systems
69 EXTENSION SYSTEMS Extension systems are zones where plates split into two or more smaller blocks that move apart. To accommodate the separation, dominantly normal faults and even open fissures lead to stretching, rupture and lengthening of crustal rocks. At the same time, the lithosphere is thinned and the asthenosphere is upwelling below the necked lithosphere. Decompression during upwelling of the mantle results in partial melting. The produced basaltic magma is injected into the fissures or extruded as fissure eruptions along and on either side of the splitting linear region (graben and rifts). This mechanism, coeval lithospheric stretching and accretion of buoyant magma, is called rifting. It is called seafloor spreading once a rifted region becomes a plate boundary that creates new oceanic lithosphere as plates diverge from one another. The spreading centres shape elevated morphological forms, the mid-oceanic ridges, because magma and young, thin oceanic lithosphere are buoyant. Divergent plate boundaries are some of the most active volcanic zones on the Earth. Seafloor spreading is so important that it has created more than half of the Earth’s surface during the past 200 Ma. Since the new continents drift away from the locus of extension, they escape further deformation and marine sedimentation seals relict structures of the early rift on either side of the new ocean. These two sides are passive continental margins. The dominant stress field is extension. Bulk lithospheric rheologies control the development of large- scale extensional -
Insights from Cordilleran Metamorphic Core Complexes
Solid Earth, 8, 199–215, 2017 www.solid-earth.net/8/199/2017/ doi:10.5194/se-8-199-2017 © Author(s) 2017. CC Attribution 3.0 License. Rheological transitions in the middle crust: insights from Cordilleran metamorphic core complexes Frances J. Cooper1, John P. Platt2, and Whitney M. Behr3 1School of Earth Sciences, University of Bristol, Bristol BS8 1RJ, UK 2Department of Earth Sciences, University of Southern California, Los Angeles, CA 90089, USA 3School of Geological Sciences, Jackson School of Geosciences, University of Texas at Austin, Austin, TX 78712, USA Correspondence to: Frances J. Cooper ([email protected]) Received: 15 September 2016 – Discussion started: 4 October 2016 Revised: 17 January 2017 – Accepted: 24 January 2017 – Published: 21 February 2017 Abstract. High-strain mylonitic rocks in Cordilleran meta- perposed sequences of microstructures reflecting progressive morphic core complexes reflect ductile deformation in the exhumation, cooling, and strain localization. The LDT is not middle crust, but in many examples it is unclear how always exhumed, or it may be obscured by later deformation, these mylonites relate to the brittle detachments that over- but in the Whipple Mountains, it can be directly observed lie them. Field observations, microstructural analyses, and where high-strain mylonites captured from the middle crust thermobarometric data from the footwalls of three meta- depart from the brittle detachment along a mylonitic front. morphic core complexes in the Basin and Range Province, USA (the Whipple -
The Role of Magmatism in the Catalina Metamorphic Core Complex, Arizona: Insights from Integrated Thermochronology, Gravity and Aeromagnetic Data
Syracuse University SURFACE Earth Sciences - Dissertations College of Arts and Sciences 5-2012 The Role of Magmatism in the Catalina Metamorphic Core Complex, Arizona: Insights from Integrated Thermochronology, Gravity and Aeromagnetic Data Jessica Terrien Syracuse University Follow this and additional works at: https://surface.syr.edu/ear_etd Part of the Earth Sciences Commons Recommended Citation Terrien, Jessica, "The Role of Magmatism in the Catalina Metamorphic Core Complex, Arizona: Insights from Integrated Thermochronology, Gravity and Aeromagnetic Data" (2012). Earth Sciences - Dissertations. 28. https://surface.syr.edu/ear_etd/28 This Dissertation is brought to you for free and open access by the College of Arts and Sciences at SURFACE. It has been accepted for inclusion in Earth Sciences - Dissertations by an authorized administrator of SURFACE. For more information, please contact [email protected]. ABSTRACT The footwall of the Catalina MCC located within the Basin and Range Province has been intruded by several magmatic suites. Samples were collected from the Wilderness suite sills, the most voluminous suite. U/Pb data from zircon indicate that the Wilderness suite sills were emplaced during two separate phases of magmatism, the first at ~55 Ma and the second at ~45 Ma with additional evidence of zircon growth at <40 Ma. 40Ar/39Ar data from the Wilderness suite sills indicate that the majority of the older 40Ar/39Ar apparent ages from both potassium feldspar and mica were obtained from the structurally higher sills in the main range, and the younger 40Ar/39Ar apparent ages were obtained on samples from structurally lower sills. The potassium feldspar samples yield 40Ar/39Ar age gradients which may be the result of cooling for ~5 million years or thermal resetting by the Catalina pluton prior to exhumation along the Catalina detachment fault.