Synthesis, GC–MS, GC–MS/MS, GC–IR and Chromatographic Studies on Cathinone Derivatives Related to Methylenedioxypyrovalerone (MDPV)
Total Page:16
File Type:pdf, Size:1020Kb
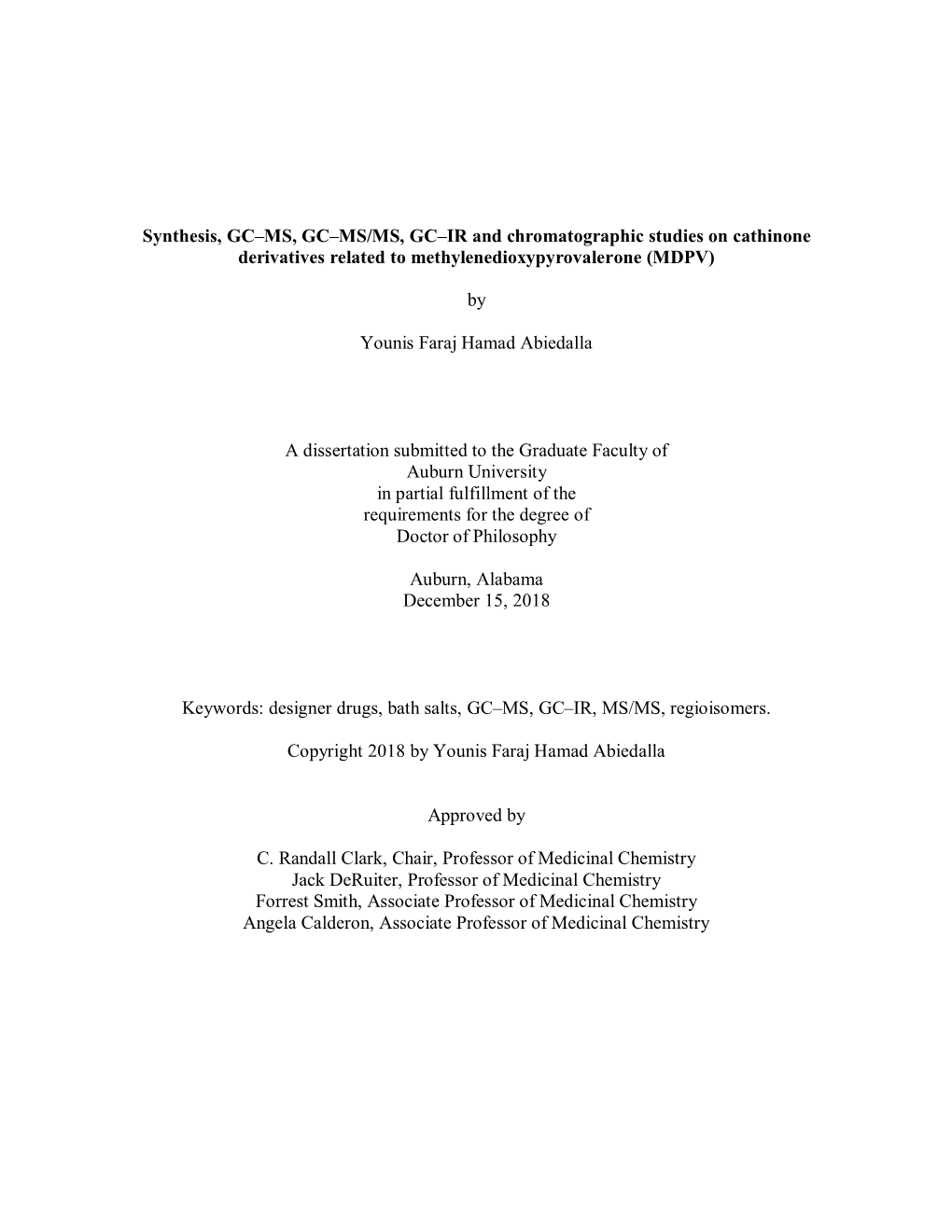
Load more
Recommended publications
-
Recommended Methods for the Identification and Analysis of Synthetic Cathinones in Seized Materialsd
Recommended methods for the Identification and Analysis of Synthetic Cathinones in Seized Materials (Revised and updated) MANUAL FOR USE BY NATIONAL DRUG ANALYSIS LABORATORIES Photo credits:UNODC Photo Library; UNODC/Ioulia Kondratovitch; Alessandro Scotti. Laboratory and Scientific Section UNITED NATIONS OFFICE ON DRUGS AND CRIME Vienna Recommended Methods for the Identification and Analysis of Synthetic Cathinones in Seized Materials (Revised and updated) MANUAL FOR USE BY NATIONAL DRUG ANALYSIS LABORATORIES UNITED NATIONS Vienna, 2020 Note Operating and experimental conditions are reproduced from the original reference materials, including unpublished methods, validated and used in selected national laboratories as per the list of references. A number of alternative conditions and substitution of named commercial products may provide comparable results in many cases. However, any modification has to be validated before it is integrated into laboratory routines. ST/NAR/49/REV.1 Original language: English © United Nations, March 2020. All rights reserved, worldwide. The designations employed and the presentation of material in this publication do not imply the expression of any opinion whatsoever on the part of the Secretariat of the United Nations concerning the legal status of any country, territory, city or area, or of its authorities, or concerning the delimitation of its frontiers or boundaries. Mention of names of firms and commercial products does not imply the endorse- ment of the United Nations. This publication has not been formally edited. Publishing production: English, Publishing and Library Section, United Nations Office at Vienna. Acknowledgements The Laboratory and Scientific Section of the UNODC (LSS, headed by Dr. Justice Tettey) wishes to express its appreciation and thanks to Dr. -
Identifying New/Emerging Psychoactive Substances at the Time of COVID-19; a Web-Based Approach
ORIGINAL RESEARCH published: 09 February 2021 doi: 10.3389/fpsyt.2020.632405 Identifying New/Emerging Psychoactive Substances at the Time of COVID-19; A Web-Based Approach Valeria Catalani 1*, Davide Arillotta 1, John Martin Corkery 1, Amira Guirguis 1,2, Alessandro Vento 3,4,5 and Fabrizio Schifano 1 1 Psychopharmacology, Drug Misuse & Novel Psychoactive Substances Research Unit, School of Life & Medical Sciences, University of Hertfordshire, Hatfield, United Kingdom, 2 Swansea University Medical School, Institute of Life Sciences 2, Swansea University, Swansea, United Kingdom, 3 Department of Mental Health, ASL Roma 2, Rome, Italy, 4 Addictions’ Observatory (ODDPSS), Rome, Italy, 5 Department of Psychology, Guglielmo Marconi University, Rome, Italy COVID-19-related disruptions of people and goods’ circulation can affect drug markets, especially for new psychoactive substances (NPSs). Drug shortages could cause a change in available NPS, with the introduction of new, unknown, substances. The aims of the current research were to use a web crawler, NPSfinder®, to identify and categorize emerging NPS discussed on a range of drug enthusiasts/psychonauts’ websites/fora at the time of the pandemic; social media for these identified NPS were screened as Edited by: well. The NPSfinder® was used here to automatically scan 24/7 a list of psychonaut Ornella Corazza, University of Hertfordshire, websites and NPS online resources. The NPSs identified in the time frame between United Kingdom January and August 2020 were searched in both the European Monitoring Center Reviewed by: for Drugs and Drug Addictions (EMCDDA)/United Nations Office on Drugs and Crime Simona Zaami, Sapienza University of Rome, Italy (UNODC) databases and on social media (Facebook, Twitter, Instagram, Pinterest, Laura Hondebrink, and YouTube) as well, with a content qualitative analysis having been carried out on University Medical Center reddit.com. -
Precursors and Chemicals Frequently Used in the Illicit Manufacture Of
40 INCB REPORT ON PRECURSORS 2019 • 2,5-Dimethoxybenzaldehyde, a precursor for 2,5-dimethoxyamphetamine (DMA), brolamfetamine IV. Article 13 of the (DOB) and the 2C-series of controlled psychotropic substances, as well as for new psychoactive substances, 1988 Convention as reported by the Netherlands (5 kg) and Belgium (1 kg). a complementary tool in addressing • 4-Methoxy-P-2-P, a precursor of para-methoxy- alpha-methylphenethylamine (PMA) and para- illicit drug methoxymethylamphetamine (PMMA), reported by Spain (52 kg). manufacture 226. Through PICS, incidents involving 2-bromo- 4’-chloropropiophenone, a precursor of various 4-chloro- 229. The clandestine manufacture of narcotic drugs and substituted cathinone derivatives, such as 4-CMC psychotropic substances, new psychoactive substances and (clephedrone), were communicated. Luxembourg seized precursors is not possible without the input of chemicals, 500 kg of the substance in August 2018. The consignment materials and equipment. While the control of chemicals was confiscated because both the supplier and the con- has long been a focus of the authorities worldwide, pursu- signee were already known in connection with shipments ant to the provisions in article 12 of the 1988 Convention, of other precursors of new psychoactive substances. It much less attention has been given to equipment and originated in India, transited Qatar, Luxembourg and materials and article 13 of that Convention, which pro- Germany and was destined for a consignee in Poland. A vides a basis for international action and cooperation in consignment of 300 kg of the substance was confiscated by such control efforts (see box 5). customs authorities in Germany in December 2018. -
Ce4less.Com Ce4less.Com Ce4less.Com Ce4less.Com Ce4less.Com Ce4less.Com Ce4less.Com
Hallucinogens And Dissociative Drug Use And Addiction Introduction Hallucinogens are a diverse group of drugs that cause alterations in perception, thought, or mood. This heterogeneous group has compounds with different chemical structures, different mechanisms of action, and different adverse effects. Despite their description, most hallucinogens do not consistently cause hallucinations. The drugs are more likely to cause changes in mood or in thought than actual hallucinations. Hallucinogenic substances that form naturally have been used worldwide for millennia to induce altered states for religious or spiritual purposes. While these practices still exist, the more common use of hallucinogens today involves the recreational use of synthetic hallucinogens. Hallucinogen And Dissociative Drug Toxicity Hallucinogens comprise a collection of compounds that are used to induce hallucinations or alterations of consciousness. Hallucinogens are drugs that cause alteration of visual, auditory, or tactile perceptions; they are also referred to as a class of drugs that cause alteration of thought and emotion. Hallucinogens disrupt a person’s ability to think and communicate effectively. Hallucinations are defined as false sensations that have no basis in reality: The sensory experience is not actually there. The term “hallucinogen” is slightly misleading because hallucinogens do not consistently cause hallucinations. 1 ce4less.com ce4less.com ce4less.com ce4less.com ce4less.com ce4less.com ce4less.com How hallucinogens cause alterations in a person’s sensory experience is not entirely understood. Hallucinogens work, at least in part, by disrupting communication between neurotransmitter systems throughout the body including those that regulate sleep, hunger, sexual behavior and muscle control. Patients under the influence of hallucinogens may show a wide range of unusual and often sudden, volatile behaviors with the potential to rapidly fluctuate from a relaxed, euphoric state to one of extreme agitation and aggression. -
Forensic Science
Anal. Chem. 1999, 71, 235R-255R Forensic Science T. A. Brettell* Forensic Science Bureau, New Jersey State Police, Box 7068, West Trenton, New Jersey 08625 K. Inman California Department of Justice DNA Laboratory, 626 Bancroft Way, Berkeley, California 94710 N. Rudin² 1563 Solano Ave. #506, Berkeley, California 94707 R. Saferstein³ Box 1334, Mount Laurel, New Jersey 08054 Review Contents Canadian Society of Forensic Science, Journal of Forensic Identifica- Drugs and Poisons 235R tion, Forensic Science Review, Analytical Toxicology, Electrophoresis, Ethanol and Volatiles 235R and BioTechniques, as well as Chemical Abstracts Selects: Forensic Cannabinoids 236R Chemistry. Our survey encompasses the period from January 1997 Morphine and Related Narcotics 237R through December 1998. Because of the normal delays in the Cocaine 237R abstraction of journal articles by Chemical Abstracts, some work Amphetamines 238R covering this period will inadvertently be omitted. Hopefully these Barbiturates 239R references will be included in the next biennial review. Benzodiazepines 239R The format selected for this survey divides coverage into three Miscellaneous Drugs and Poisons 239R distinct areas: drug and poisons, forensic DNA analysis, and trace General Procedures 241R Forensic DNA Analysis 242R evidence. Within the scope of each of the areas, articles have been DNA Extraction and Quantitation 242R selected to describe current forensic science practices in analytical Restriction Fragment Length Polymorphisms 242R chemistry and to outline relevant forensic science research Amplified Fragment Length Polymorphisms 242R interests. To keep our discussion concise and meaningful, we have Short Tandem Repeats 242R limited our survey to drugs regulated under the United States Gender Identification 243R Controlled Substances Act, ethanol, and common poisons. -
Newer Unregulated Drugs Look-Up Table
Newer Unregulated Drugs Look-up Table List Name Chemical Name/AKA Type of drug Notes Stimulant Regulation under MDA (Sch. 1 or TCDO) Stimulant/Hallucinogen Regulation under MDA (Sch. 2-5) Hallucinogen Regulated by PSA Depressant Exempt Cannabinoid Uncertain/requires clarification 1P-LSD 1-propionyl-lysergic acid diethylamide Hallucinogen An LSD analogue that side-stepped MDA and was on sale as an NPS; now covered by the PSA. 2-AI 2-Aminoindane Stimulant, amphetamine analogue Reported in the UK in 2011 by the Forensic Early 2-MAI N-methyl-2-Aminoindane Warning System (FEWS). Had been on sale via number MMAI of online stores; covered by PSA. 2-MeO-ketamine Methoxyketamine Related to methoxetamine so a relative Believed to have been made a CD at the same time as Methoxieticyclidine of ketamine – i.e. a dissassociative Methoxetamine anaesthetic hallucinogen 2C-B-BZP (1-(4-bromo-2,5- Piperazine family; stimulant Class B dimethoxybenzyl)piperazine) 2-DPMP Desoxypipadrol stimulant Strong and long acting stimulant; reported duration of 2-diphenylmethylpiperidine effect 24-28hrs or more and effective at very low doses. Had been on sale in the UK and cropped up in branded “Ivory Wave” and in other compounds. Linked to fatalities. Class B, Sch1. 2-NE1 APICA Synthetic cannabinoid receptor agonist 3rd generation SCRA. Covered by PSA SDB-001 N-(1-adamantyl)-1-pentyl-1H-indole-3- carboxamide 3-FPM Phenzacaine Stimulant, euphoriants Sibling of the controlled drug Phenmetrazine. Emerged PAL-593 2015. Covered by PSA 2-(3-fluorophenyl)-3-methylmorpholine 3-hydroxyphenazepam Benzo, GABA-nergic PSA 3-MeO-PCE (3-methoxyeticyclidine) Related to methoxetamine so a relative Probably regulated under the same clause that made of ketamine – i.e. -
A Chemical Analysis Examining the Pharmacology of Novel Psychoactive Substances Freely Available Over the Internet and Their Impact on Public (Ill) Health
Open Access Research A chemical analysis examining the pharmacology of novel psychoactive substances freely available over the internet and their impact on public (ill) health. Legal highs or illegal highs? Tammy C Ayres,1,2 John W Bond3 To cite: Ayres TC, Bond JW. ABSTRACT ARTICLE SUMMARY A chemical analysis Objectives: Public Health England aims to improve the examining the pharmacology nation’s health and acknowledges that unhealthy Article focus of novel psychoactive lifestyles, which include drug use, undermine society’s - substances freely available To analyse the chemical composition of health and well-being. Recreational drug use has over the internet and their substances bought over the internet, including impact on public (ill)health. changed to include a range of substances sold as the legality of the active ingredients and if Legal highs or illegal highs? ‘research chemicals’ but known by users as ‘legal highs’ products differ between retailers. BMJ Open 2012;2:e000977. (legal alternatives to the most popular illicit recreational - To consider the medical implications and adverse doi:10.1136/ drugs), which are of an unknown toxicity to humans and health risks associated with legal highs bought bmjopen-2012-000977 often include prohibited substances controlled under the over the internet. Misuse of Drugs Act (1971). Consequently, the long- term effects on users’ health and inconsistent, often Key messages < Prepublication history for illegal ingredients, mean that this group of drugs - The most recent examination of the composition this paper is available online. presents a serious risk to public health both now and in of ‘legal highs’, conducted 6 months after the To view these files please the future. -
Synthetic Cannabinoids & Substituted Cathinones a Local Perspective
Emerging Trends in Synthetic Drugs Anthony J. Tambasco Mansfield Division of Police Forensic Science Laboratory Midwestern Association of Forensic Scientists (MAFS) . 900 Members throughout the U.S. Midwest Region includes Ohio, Indiana, Illinois, Iowa, North Dakota, South Dakota, Minnesota, Michigan, Wisconsin. Chemistry Section Coordinator – Jillian Baker, DuPage County Laboratory, Illinois . 2013 Annual Meeting – Dayton, Ohio (September 30- October 4) February 2010 – AAFS . “Get Ready for the Cathinones and Synthetic Cannabinoids” . Laboratory Director, U.S. Customs – Chicago Michigan . Substances became controlled in October 2010, August 2011 and July 2012. Core Cannabinoid Chemical Group included in the 2012 legislation. Any other synthetic compound that is a cannabinoid receptor agonist…not listed in Schedules II – V and is not approved by the federal food and drug administration as a drug. Michigan . Non-controlled substances are being reported as such with a follow up call to the agency advising what the substance may be. Current emergency ruling covering the substituted phenethylamines are in place until July 2013. Illinois . A limited number of synthetic compounds are listed by name with five classes of synthetic cannabinoids. State’s Attorney’s Office is not pursuing analog charges. Laboratory will use literature references from two reliable sources in the absence of an available standard. Illinois . Rush cases on approval of State’s Attorney’s Office. State law prohibits the use of sampling plans, so each packet is analyzed individually. 200 gram maximum. Illinois . Traditional GC/MS methods – run times up to 45 minutes. Recent drop-off in “Bath Salt” cases. Suspected LSD cases negative for LSD have been found to contain NBOMe compounds. -
Structure-Activity Relationships of Synthetic Cathinones
Structure-Activity Relationships of Synthetic Cathinones Richard A. Glennon and Małgorzata Dukat Abstract Until recently, there was rather little interest in the structure-activity relationships (SARs) of cathinone analogs because so few agents were available and because they represented a relatively minor drug abuse problem. Most of the early SAR was formulated on the basis of behavioral (e.g., locomotor and drug discrimination) studies using rodents. With the emergence on the clandestine market in the last few years of a large number of new cathinone analogs, termed “synthetic cathinones”, and the realization that they likely act at dopamine, norepi- nephrine, and/or serotonin transporters as releasing agents (i.e., as substrates) or reuptake inhibitors (i.e., as transport blockers), it has now become possible to better examine their SAR and even their quantitative SAR (QSAR), in a more effective and systematic manner. An SAR picture is beginning to emerge, and key structural features, such as the nature of the terminal amine, the size of the α-substituent, stereochemistry, and the presence and position of aromatic substituents, are being found to impact action (i.e., as releasing agents or reuptake inhibitors) and trans- porter selectivity. Keywords DAT • Methcathinone • Monoamine transporters • NET • QSAR • SAR • SERT Contents 1 Introduction ................................................................................... 20 2 Early SAR of Cathinone ..................................................................... 23 3 The Methcathinone -
Model Novel Psychoactive Substances Economic Sanctions
Model Novel Psychoactive Substances – Economic Sanctions Package Contact information updated September 2018. For information about this document, please contact: Jon Woodruff, Senior Legislative Attorney National Alliance for Model State Drug Laws Email: [email protected] Phone: 703.836.7496 Headquarters Office: National Alliance for Model State Drug Laws (NAMSDL) 1335 North Front Street, First Floor Harrisburg, PA 17102-2629 Model Novel Psychoactive Substances – Economic Sanctions Package © 2014. This document is intended for educational purposes only and does not constitute legal advice or opinion. Headquarters Office: THE NATIONAL ALLIANCE FOR MODEL STATE DRUG LAWS. 215 Lincoln Ave. Suite 201, Santa Fe, NM 87501. 1 Table of Contents 3 Policy Statement 5 Highlights Part One 6 Model Revocation or Suspension of Business License Act Section One Court Recommendation Section Two Effect on Alcohol or Tobacco License Section Three Statutory Construction Section Four Severability Provision Section Five Effective Date Part Two 9 Model Injunctive and Other Equitable Relief Act Section One Equitable Relief Section Two Statutory Construction Section Three Severability Provision Section Four Effective Date Part Three 11 Model Nuisance Abatement Act Section One Finding of Drug-Related Nuisance Section Two Conviction for Novel Psychoactive Substance Offense Section Three Statutory Construction Section Four Severability Provision Section Five Effective Date Part Four 13 Model Mislabeled or Misbranded Products Act Section One Definitions Section Two Seizure of Products Section Three Statutory Construction Section Four Severability Provision Section Five Effective Date Appendix A 14 Additional Model Language Which May Be Used by Municipalities and Counties © 2014. This document is intended for educational purposes only and does not constitute legal advice or opinion. -
A Review of the Collision Induced Dissociation Fragmentation and the Metabolism of Synthetic Cathinone Derivatives
A review of the collision induced dissociation fragmentation and the metabolism of synthetic cathinone derivatives Nichola Cunningham A thesis submitted in fulfilment of the requirements for the degree of Master of Forensic Science (Professional Practice) In The school of Veterinary and Life Sciences Murdoch University Supervisors: Dr John Coumbaros Associate Professor James Speers Semester 1 2018 i Declaration I declare that this thesis does not contain any material submitted previously for the award of any other degree or diploma at any university or other tertiary institution. Furthermore, to the best of my knowledge, it does not contain any material previously published or written by another individual, except where due reference has been made in the text. Finally, I declare that all reported experimentations performed in this research were carried out by myself, except that any contributions by others, with whom I have worked is explicitly acknowledged. Signed: Nichola Cunningham ii Acknowledgments Firstly, I would like to thank my supervisor John Coumbaros for all of your support, feedback, and advice throughout this project. You have been an invaluable help and I am grateful for all the time you invested in my work. To Bob Mead, thank you so much for all your help with this project. You have always been a support throughout the years and this was no exception; thank you for the time and feedback you provided me along the way. Finally, to my family and friends who have supported me through this, and the entire degree, thank you! I finally made it through! iii Table of Contents Title page ...................................................................................................................................... -
COMMITTEE/SUBCOMMITTEE AMENDMENT Bill No. HB 1175 (2012) Amendment No
COMMITTEE/SUBCOMMITTEE AMENDMENT Bill No. HB 1175 (2012) Amendment No. 1 COMMITTEE/SUBCOMMITTEE ACTION ADOPTED (Y/N) ADOPTED AS AMENDED (Y/N) ADOPTED W/O OBJECTION (Y/N) FAILED TO ADOPT (Y/N) WITHDRAWN (Y/N) OTHER 1 Committee/Subcommittee hearing bill: Criminal Justice 2 Subcommittee 3 Representative Ingram offered the following: 4 5 Amendment 6 Remove lines 147-236 and insert: 7 84. Naphyrone (naphthylpyrovalerone). 8 85. N-N-Dimethyl-3,4-methylenedioxycathinone. 9 86. N-N-Diethyl-3,4-methylenedioxycathinone. 10 87. 3,4-methylenedioxy-propiophenone. 11 88. 2-Bromo-3,4-Methylenedioxypropiophenone. 12 89. 3,4-methylenedioxy-propiophenone-2-oxime. 13 90. N-Acetyl-3,4-methylenedioxycathinone. 14 91. N-Acetyl-N-Methyl-3,4-Methylenedioxycathinone. 15 92. N-Acetyl-N-Ethyl-3,4-Methylenedioxycathinone. 16 93. Bromomethcathinone. 17 94. Buphedrone (alpha-methylamino-butyrophenone). 18 95. Eutylone (beta-Keto-Ethylbenzodioxolylbutanamine). 19 96. Dimethylcathinone. 964151 - h1175-line147.docx Published On: 1/13/2012 6:13:24 PM Page 1 of 4 COMMITTEE/SUBCOMMITTEE AMENDMENT Bill No. HB 1175 (2012) Amendment No. 1 20 97. Dimethylmethcathinone. 21 98. Pentylone (beta-Keto-Methylbenzodioxolylpentanamine). 22 99. (MDPPP) 3,4-Methylenedioxy-alpha-pyrrolidinopropiophenone. 23 100. (MDPBP) 3,4-Methylenedioxy-alpha-pyrrolidinobutiophenone. 24 101. Methoxypyrrolidinopropiophenone (MOPPP). 25 102. Methylpyrrolidinohexiophenone (MPHP). 26 103. Benzocyclidine (BCP) or benzothiophenylcyclohexylpiperidine 27 (BTCP). 28 104. Fluoromethylaminobutyrophenone (F-MABP). 29 105. Methoxypyrrolidinobutyrophenone (MeO-PBP). 30 106. Ethylpyrrolidinobutyrophenone (Et-PBP). 31 107. 3-Methyl-4-Methoxymethcathinone (3-Me-4-MeO-MCAT). 32 108. Methylethylaminobutyrophenone (Me-EABP) 33 109. Methylaminobutyrophenone (MABP). 34 110. Pyrrolidinopropiophenone. 35 111. Pyrrolidinobutiophenone (PBP). 36 112. Pyrrolidinovalerophenone (PVP).