Squaring the Circle: Ancient Problems and Modern Solutions
Total Page:16
File Type:pdf, Size:1020Kb
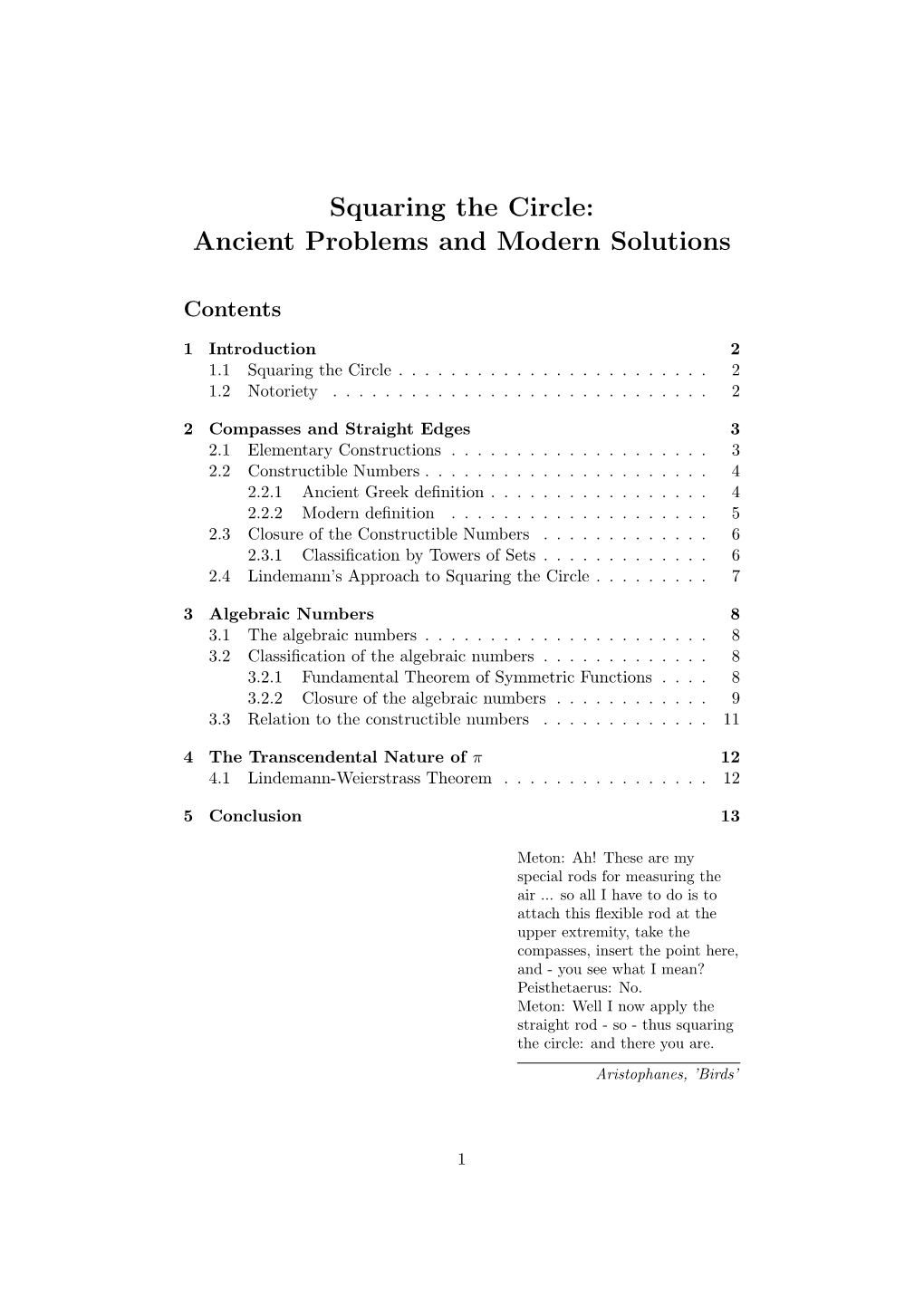
Load more
Recommended publications
-
Arnold Sommerfeld in Einigen Zitaten Von Ihm Und Über Ihn1
K.-P. Dostal, Arnold Sommerfeld in einigen Zitaten von ihm und über ihn Seite 1 Karl-Peter Dostal, Arnold Sommerfeld in einigen Zitaten von ihm und über ihn1 Kurze biographische Bemerkungen Arnold Sommerfeld [* 5. Dezember 1868 in Königsberg, † 26. April 1951 in München] zählt neben Max Planck, Albert Einstein und Niels Bohr zu den Begründern der modernen theoretischen Physik. Durch die Ausarbeitung der Bohrschen Atomtheorie, als Lehrbuchautor (Atombau und Spektrallinien, Vorlesungen über theoretische Physik) und durch seine „Schule“ (zu der etwa die Nobelpreisträger Peter Debye, Wolfgang Pauli, Werner Heisenberg und Hans Bethe gehören) sorgte Sommerfeld wie kein anderer für die Verbreitung der modernen Physik.2 Je nach Auswahl könnte Sommerfeld [aber] nicht nur als theoretischer Physiker, sondern auch als Mathematiker, Techniker oder Wissenschaftsjournalist porträtiert werden.3 Als Schüler der Mathematiker Ferdinand von Lindemann, Adolf Hurwitz, David Hilbert und Felix Klein hatte sich Sommerfeld zunächst vor allem der Mathematik zugewandt (seine erste Professur: 1897 - 1900 für Mathematik an der Bergakademie Clausthal). Als Professor an der TH Aachen von 1900 - 1906 gewann er zunehmendes Interesse an der Technik. 1906 erhielt er den seit Jahren verwaisten Lehrstuhl für theoretische Physik in München, an dem er mit wenigen Unterbrechungen noch bis 1940 (und dann wieder ab 19464) unterrichtete. Im Gegensatz zur etablierten Experimen- talphysik war die theoretische Physik anfangs des 20. Jh. noch eine junge Disziplin. Sie wurde nun zu -
Squaring the Circle a Case Study in the History of Mathematics the Problem
Squaring the Circle A Case Study in the History of Mathematics The Problem Using only a compass and straightedge, construct for any given circle, a square with the same area as the circle. The general problem of constructing a square with the same area as a given figure is known as the Quadrature of that figure. So, we seek a quadrature of the circle. The Answer It has been known since 1822 that the quadrature of a circle with straightedge and compass is impossible. Notes: First of all we are not saying that a square of equal area does not exist. If the circle has area A, then a square with side √A clearly has the same area. Secondly, we are not saying that a quadrature of a circle is impossible, since it is possible, but not under the restriction of using only a straightedge and compass. Precursors It has been written, in many places, that the quadrature problem appears in one of the earliest extant mathematical sources, the Rhind Papyrus (~ 1650 B.C.). This is not really an accurate statement. If one means by the “quadrature of the circle” simply a quadrature by any means, then one is just asking for the determination of the area of a circle. This problem does appear in the Rhind Papyrus, but I consider it as just a precursor to the construction problem we are examining. The Rhind Papyrus The papyrus was found in Thebes (Luxor) in the ruins of a small building near the Ramesseum.1 It was purchased in 1858 in Egypt by the Scottish Egyptologist A. -
Squaring the Circle in Elliptic Geometry
Rose-Hulman Undergraduate Mathematics Journal Volume 18 Issue 2 Article 1 Squaring the Circle in Elliptic Geometry Noah Davis Aquinas College Kyle Jansens Aquinas College, [email protected] Follow this and additional works at: https://scholar.rose-hulman.edu/rhumj Recommended Citation Davis, Noah and Jansens, Kyle (2017) "Squaring the Circle in Elliptic Geometry," Rose-Hulman Undergraduate Mathematics Journal: Vol. 18 : Iss. 2 , Article 1. Available at: https://scholar.rose-hulman.edu/rhumj/vol18/iss2/1 Rose- Hulman Undergraduate Mathematics Journal squaring the circle in elliptic geometry Noah Davis a Kyle Jansensb Volume 18, No. 2, Fall 2017 Sponsored by Rose-Hulman Institute of Technology Department of Mathematics Terre Haute, IN 47803 a [email protected] Aquinas College b scholar.rose-hulman.edu/rhumj Aquinas College Rose-Hulman Undergraduate Mathematics Journal Volume 18, No. 2, Fall 2017 squaring the circle in elliptic geometry Noah Davis Kyle Jansens Abstract. Constructing a regular quadrilateral (square) and circle of equal area was proved impossible in Euclidean geometry in 1882. Hyperbolic geometry, however, allows this construction. In this article, we complete the story, providing and proving a construction for squaring the circle in elliptic geometry. We also find the same additional requirements as the hyperbolic case: only certain angle sizes work for the squares and only certain radius sizes work for the circles; and the square and circle constructions do not rely on each other. Acknowledgements: We thank the Mohler-Thompson Program for supporting our work in summer 2014. Page 2 RHIT Undergrad. Math. J., Vol. 18, No. 2 1 Introduction In the Rose-Hulman Undergraduate Math Journal, 15 1 2014, Noah Davis demonstrated the construction of a hyperbolic circle and hyperbolic square in the Poincar´edisk [1]. -
Pappus of Alexandria: Book 4 of the Collection
Pappus of Alexandria: Book 4 of the Collection For other titles published in this series, go to http://www.springer.com/series/4142 Sources and Studies in the History of Mathematics and Physical Sciences Managing Editor J.Z. Buchwald Associate Editors J.L. Berggren and J. Lützen Advisory Board C. Fraser, T. Sauer, A. Shapiro Pappus of Alexandria: Book 4 of the Collection Edited With Translation and Commentary by Heike Sefrin-Weis Heike Sefrin-Weis Department of Philosophy University of South Carolina Columbia SC USA [email protected] Sources Managing Editor: Jed Z. Buchwald California Institute of Technology Division of the Humanities and Social Sciences MC 101–40 Pasadena, CA 91125 USA Associate Editors: J.L. Berggren Jesper Lützen Simon Fraser University University of Copenhagen Department of Mathematics Institute of Mathematics University Drive 8888 Universitetsparken 5 V5A 1S6 Burnaby, BC 2100 Koebenhaven Canada Denmark ISBN 978-1-84996-004-5 e-ISBN 978-1-84996-005-2 DOI 10.1007/978-1-84996-005-2 Springer London Dordrecht Heidelberg New York British Library Cataloguing in Publication Data A catalogue record for this book is available from the British Library Library of Congress Control Number: 2009942260 Mathematics Classification Number (2010) 00A05, 00A30, 03A05, 01A05, 01A20, 01A85, 03-03, 51-03 and 97-03 © Springer-Verlag London Limited 2010 Apart from any fair dealing for the purposes of research or private study, or criticism or review, as permitted under the Copyright, Designs and Patents Act 1988, this publication may only be reproduced, stored or transmitted, in any form or by any means, with the prior permission in writing of the publishers, or in the case of reprographic reproduction in accordance with the terms of licenses issued by the Copyright Licensing Agency. -
Greek Mathematics Recovered in Books 6 and 7 of Clavius’ Geometria Practica
Introduction – Clavius and Geometria Practica Book 6 and Greek approaches to duplication of the cube Book 7 and squaring the circle via the quadratrix Conclusions Greek Mathematics Recovered in Books 6 and 7 of Clavius’ Geometria Practica John B. Little Department of Mathematics and CS College of the Holy Cross June 29, 2018 Greek Mathematics in Clavius Introduction – Clavius and Geometria Practica Book 6 and Greek approaches to duplication of the cube Book 7 and squaring the circle via the quadratrix Conclusions I’ve always been interested in the history of mathematics (in addition to my nominal specialty in algebraic geometry/computational methods/coding theory, etc.) Want to be able to engage with original texts on their own terms – you might recall the talks on Apollonius’s Conics I gave at the last Clavius Group meeting at Holy Cross (two years ago) So, I’ve been taking Greek and Latin language courses in HC’s Classics department The subject for today relates to a Latin-to-English translation project I have recently begun – working with the Geometria Practica of Christopher Clavius, S.J. (1538 - 1612, CE) Greek Mathematics in Clavius Introduction – Clavius and Geometria Practica Book 6 and Greek approaches to duplication of the cube Book 7 and squaring the circle via the quadratrix Conclusions Overview 1 Introduction – Clavius and Geometria Practica 2 Book 6 and Greek approaches to duplication of the cube 3 Book 7 and squaring the circle via the quadratrix 4 Conclusions Greek Mathematics in Clavius Introduction – Clavius and Geometria Practica Book 6 and Greek approaches to duplication of the cube Book 7 and squaring the circle via the quadratrix Conclusions Clavius’ Principal Mathematical Textbooks Euclidis Elementorum, Libri XV (first ed. -
Math 109: Mathematics for Design
MATH 109: MATHEMATICS FOR DESIGN SUMMARY OF GOALS FOR THE COURSE In our contemporary culture the dialogue between math and art, while sometimes strained by Course details misunderstandings, is a dynamic and living one. Art Time: MWF 10:20 – 11:30am continues to inspire and inform mathematical Place: Mon, Wed PPHAC 235 thinking, and mathematics helps artists develop Fri PPHAC 112 additional insight when reasoning about the content and structure of their work. The tools of mathematics Instructor: Kevin Hartshorn also aid in the construction of conceptual Office: PPHAC 215 frameworks that are useful in all aspects of life. Hours: Mon/Wed 2:30-3:30pm, Tue/Thu 8:30-9:30am, This course will introduce students to ideas in or by appointment mathematical thinking that are related to artistic considerations. Students will need to show e-mail: [email protected] proficiency with some mathematical ideas and then Web: https://sites.google.com/a/moravian.edu/ apply those ideas in creating their own works of art. math-109-spring-2015 In the process, students will also be called to analyze Text: Squaring the Circle: Geometry in Art existing artwork with a mathematical eye. In this and Architecture, by Paul Calter way, students will be provided a new tool to use in their approach to art and aesthetics. KEY IDEAS FOR THIS COURSE Each assignment and class discussion will be aimed at expanding on these key notions: • Mathematics and mathematical thinking involve a creative effort, not just rote memorization. • There is a rich and complex connection between mathematics and art. • Very basic mathematical concepts can be used to solve seemingly complex real-world problems. -
Ruler and Compass Constructions and Abstract Algebra
Ruler and Compass Constructions and Abstract Algebra Introduction Around 300 BC, Euclid wrote a series of 13 books on geometry and number theory. These books are collectively called the Elements and are some of the most famous books ever written about any subject. In the Elements, Euclid described several “ruler and compass” constructions. By ruler, we mean a straightedge with no marks at all (so it does not look like the rulers with centimeters or inches that you get at the store). The ruler allows you to draw the (unique) line between two (distinct) given points. The compass allows you to draw a circle with a given point as its center and with radius equal to the distance between two given points. But there are three famous constructions that the Greeks could not perform using ruler and compass: • Doubling the cube: constructing a cube having twice the volume of a given cube. • Trisecting the angle: constructing an angle 1/3 the measure of a given angle. • Squaring the circle: constructing a square with area equal to that of a given circle. The Greeks were able to construct several regular polygons, but another famous problem was also beyond their reach: • Determine which regular polygons are constructible with ruler and compass. These famous problems were open (unsolved) for 2000 years! Thanks to the modern tools of abstract algebra, we now know the solutions: • It is impossible to double the cube, trisect the angle, or square the circle using only ruler (straightedge) and compass. • We also know precisely which regular polygons can be constructed and which ones cannot. -
50 Mathematical Ideas You Really Need to Know
50 mathematical ideas you really need to know Tony Crilly 2 Contents Introduction 01 Zero 02 Number systems 03 Fractions 04 Squares and square roots 05 π 06 e 07 Infinity 08 Imaginary numbers 09 Primes 10 Perfect numbers 11 Fibonacci numbers 12 Golden rectangles 13 Pascal’s triangle 14 Algebra 15 Euclid’s algorithm 16 Logic 17 Proof 3 18 Sets 19 Calculus 20 Constructions 21 Triangles 22 Curves 23 Topology 24 Dimension 25 Fractals 26 Chaos 27 The parallel postulate 28 Discrete geometry 29 Graphs 30 The four-colour problem 31 Probability 32 Bayes’s theory 33 The birthday problem 34 Distributions 35 The normal curve 36 Connecting data 37 Genetics 38 Groups 4 39 Matrices 40 Codes 41 Advanced counting 42 Magic squares 43 Latin squares 44 Money mathematics 45 The diet problem 46 The travelling salesperson 47 Game theory 48 Relativity 49 Fermat’s last theorem 50 The Riemann hypothesis Glossary Index 5 Introduction Mathematics is a vast subject and no one can possibly know it all. What one can do is explore and find an individual pathway. The possibilities open to us here will lead to other times and different cultures and to ideas that have intrigued mathematicians for centuries. Mathematics is both ancient and modern and is built up from widespread cultural and political influences. From India and Arabia we derive our modern numbering system but it is one tempered with historical barnacles. The ‘base 60’ of the Babylonians of two or three millennia BC shows up in our own culture – we have 60 seconds in a minute and 60 minutes in an hour; a right angle is still 90 degrees and not 100 grads as revolutionary France adopted in a first move towards decimalization. -
Appendix I: on the Early History of Pi
Appendix I: On the Early History of Pi Our earliest source on 7r is a problem from the Rhind mathematical papyrus, one of the few major sources we have for the mathematics of ancient Egypt. Although the material on the papyrus in its present form comes from about 1550 B.C. during the Middle Kingdom, scholars believe that the mathematics of the document originated in the Old Kingdom, which would date it perhaps to 1900 B.C. The Egyptian source does not state an explicit value for 7r, but tells, instead, how to compute the area of a circle as the square of eight-ninths of the diameter. (One is reminded of the 'classical' problem of squaring the circle.) Quite different is the passage in I Kings 7, 23 (repeated in II Chronicles 4, 21), which clearly implies that one should multiply the diameter of a circle by 3 to find its circumference. It is worth noting, however, that the Egyptian and the Biblical texts talk about what are, a priori, different concepts: the diameter being regarded as known in both cases, the first text gives a factor used to produce the area of the circle and the other gives (by implication) a factor used to produce the circumference.1 Also from the early second millenium are Babylonian texts from Susa. In the mathematical cuneiform texts the standard factor for multiplying the diameter to produce the circumference is 3, but, according to some interpre tations, the Susa texts give a value of 31.2 Another source of ancient values of 7r is the class of Indian works known as the Sulba Sfitras, of which the four most important, and oldest are Baudhayana, .Apastamba, Katyayana and Manava (resp. -
Arxiv:1908.01202V1 [Math.GM] 3 Aug 2019 113 of Π Correct to 6 Decimal Places
SQUARE THE CIRCLE IN ONE MINUTE HÙNG VIÊ. T CHU ABSTRACT. Since squaring the circle was proved to be impossible, mathematicians have picked up a new hobby to square the circle ’approximately’. The most famous work is due to Ramanujan who gave a construction correct to 8 decimal places. In his book Mathographics, Dixon gave constructions correct to 3 decimal places and stated that his method was quite complicated. In this paper, we improve Dixon’s method then give a new construction correct to 9 decimal places, a new record so far. 1. HISTORY Squaring the circle is a problem proposed by ancient geometers who asked whether it was possible to construct a square which has the same area with a given circle using only rulers and compasses. The method is called ruler-and-compass construction or classical construction. Nowadays, we know that this task is impossible, but the problem of squaring the circle had such a long history that many circle-squarers attempted and failed. Because it is possible to approximate the area of a circle with inscribing regular polygons to any degree of precision, and polygons can also be squared, it is reasonable to expect that a circle can be squared. While many Greek mathematicians and philoso- phers including Anaxagoras, Hippocrates of Chios, and Oenopides attempted a solution, mathematicians after the 16th century tried to prove its impossibility. However, it was not until the 19th century that considerable progress was made. In 1837, Pierre Wantzel [5] proved that lengths that can be constructed must be the zero of a polynomial. -
The Ubiquity of Phi in Human Culture & the Natural World
John Carroll University Carroll Collected Masters Essays Master's Theses and Essays 2020 THE UBIQUITY OF PHI IN HUMAN CULTURE & THE NATURAL WORLD Jennifer Bressler Follow this and additional works at: https://collected.jcu.edu/mastersessays Part of the Mathematics Commons THE UBIQUITY OF PHI IN HUMAN CULTURE & THE NATURAL WORLD An Essay Submitted to the Office of Graduate Studies College of Arts & Sciences of John Carroll University In Partial Fulfillment of the Requirements For the Degree of Master of Arts By Jennifer L. Bressler 2020 Table of Contents I. Introduction…………………………………………………………………………. 2 II. The Early Greeks…………………………………………………………………… 4 III. Algebraic Properties of the Golden Ratio………………………………………….. 11 IV. The Golden Rectangle…………………………………………………….……….. 20 V. Architecture & Design……………………………………………………………… 22 VI. Art………………………………………………………………………………….. 30 VII. Music……………………………………………………………………………….. 38 VIII. The Natural World………………………………………………………………….. 43 IX. Human Anatomy…………………………………………………………………… 52 X. Geometry…………………………………………………………………………… 56 XI. Conclusion……………………………………………………………………………65 1 I. INTRODUCTION What do rabbit breeding, tornadoes, the Chambered Nautilus, a pentagram, the rhythm of a heartbeat, apple seeds, the shape of a credit card, a pinecone, the human ear, DaVinci’s Last Supper, the structure of DNA, a light switch cover, and the structure of galaxies all have in common? Each relates to an extraordinary ratio that is highly efficient in nature, profoundly attractive to the human eye, and some claim, even divinely inspired. This special ratio is referred to as the “Golden Ratio” and is also known as the divine proportion, golden section, and golden mean. The Golden Ratio has a constant numeric value called “phi” (pronounced “FEE,” or “FI”) which is thought to be the most beautiful and astounding of all numbers. -
Delian Problem (Duplication/Doubling of the Cube)
HPS 322 Michael White History of Science Fall semester, 2013 The Delian Problem (Duplication/Doubling of the Cube) There are lots of (conflicting) stories about the so-called Delian Problem. But the gist of these stories are that the citizens of the Greek island of Delos were afflicted by the plague (alternatively, by internal political unrest) and asked the oracle of the god Apollo what they could do to alleviate the situation. The oracle said that they should construct an altar to Apollo in their temple that was twice the size of the altar, in the shape of a regular cube, that they already had. They tried doubling the side of the cube, but that didn’t work (the plague, or political conflict, continued unabated). So the Delians eventually decided (with the advice of Plato?) that they should construct a cubical altar with twice the volume of the old one. (Plutarch later [1st- 2nd centuries A.D.] maintains that Plato interpreted the oracle’s response in a metaphorical or ‘demythologized’ way–as a general injunction to the Delians to spend their time studying geometry rather than fighting with one another.) Interpreted literally, then the Delian Problem is the following: given a cube of side a, to find the linear distance x such that a cube of side x is twice the volume of a cube of side a. In algebraic notation (which the Greeks did not have): Given a, to find the length x such that x33 = 2a . According to ancient reports, the mathematician Hippocrates of Chios had, in the 5th century B.C., ‘reduced’ the Delian Problem of the Duplication of the Cube to the following problem: given two line segments of length a and b, to find the line segments of length x and y in continuing geometric proportion between a and b.