Reservoir Characterization of the Bone Spring and Wolfcamp
Total Page:16
File Type:pdf, Size:1020Kb
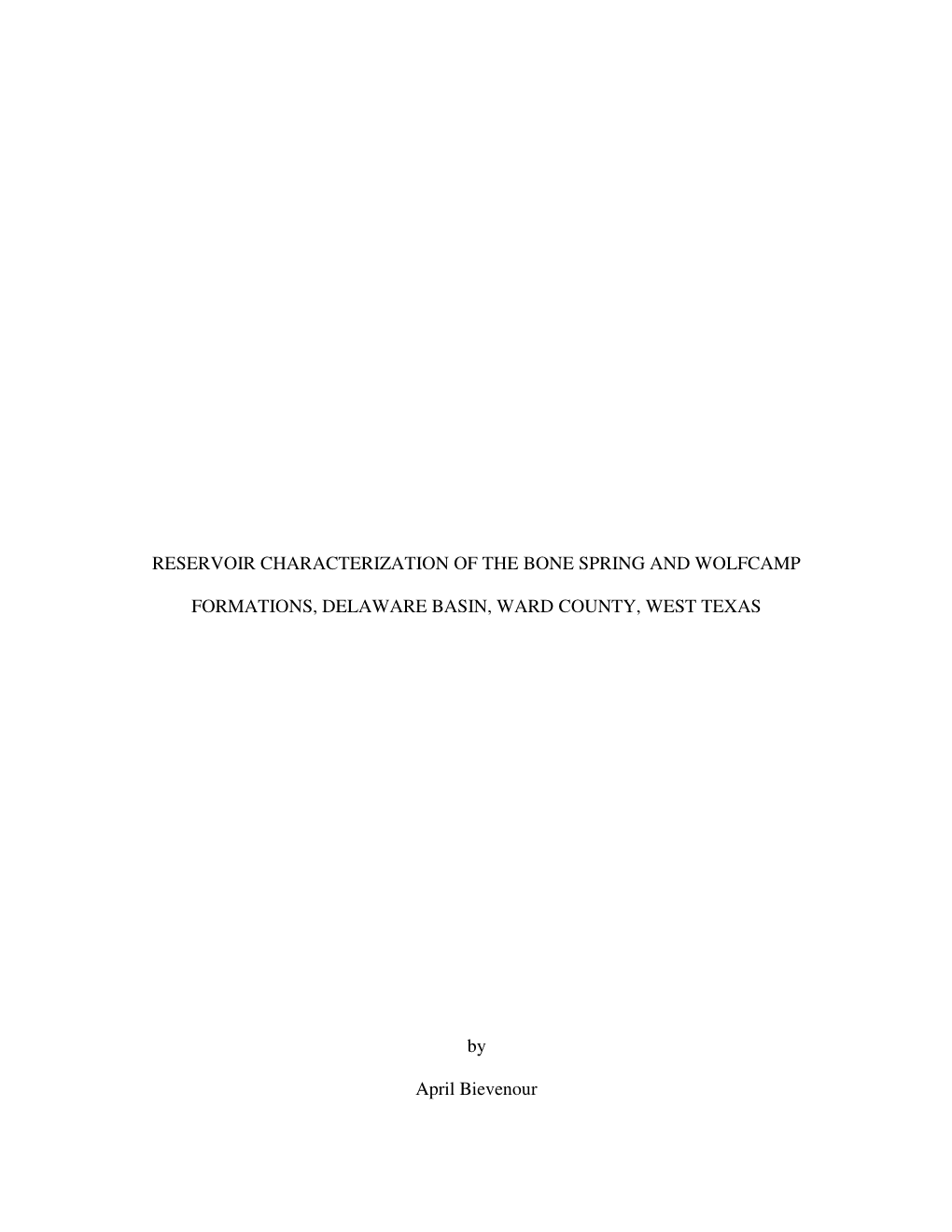
Load more
Recommended publications
-
A Few Months-Old Storm-Generated Turbidite Deposited in the Capbreton Canyon (Bay of Biscay, SW France)
Geo-Marine Letters DOI 10.1007/s003670100077 A few months-old storm-generated turbidite deposited in the Capbreton Canyon (Bay of Biscay, SW France) T. Mulder( ) · O. Weber · P. Anschutz · F. J. Jorissen · J.-M. Jouanneau T. Mulder · O. Weber · P. Anschutz · F.J. Jorissen · J.-M. Jouanneau Département de Géologie et Océanographie, UMR 5805 EPOC, Université Bordeaux I, Avenue des Facultés, 33405 Talence cedex, France E-mail: [email protected] Received: 22 January 2001 / Revision accepted: 20 July 2001 / Published online: Abstract. A gravity core taken in the canyon of Capbreton shows a succession of sedimentary facies which can be interpreted as three superimposed Bouma sequences. The turbiditic sequences are covered by an oxidised layer which contains live benthic foraminiferal faunas indicating a reprisal of hemipelagic deposition. Activities of 234 Th and 210 Pb suggest that the most recent turbidite was deposited between early December 1999 and mid-January 2000. During this period, the most probable natural event able to trigger a turbidity current was the violent storm which affected the French Atlantic coast on 27 December 1999. The turbidity current could have been caused by a sediment failure due to an excess in pore pressure generated by the storm waves, an increase of the littoral drift, or the dissipation of the along-coast water bulge through the canyon. This sub-Recent turbidite shows that the canyon experiences modern gravity processes, despite the lack of a direct connection with a major sediment source. Introduction Although turbidity currents and turbidites have been known for half a century (Kuenen 1951, 1952a, 1952b, 1953, 1959; Bouma 1962), much controversy still exists concerning the processes of deposition (Kneller and Branney 1995; Shanmugam 1997, 2000; Mulder and Alexander 2001), their origin (Mulder and Cochonat 1996) and their recognition. -
Pennsylvanian Minturn Formation, Colorado, U.S.A
Journal of Sedimentary Research, 2008, v. 78, 0–0 Research Articles DOI: 10.2110/jsr.2008.052 DEPOSITS FROM WAVE-INFLUENCED TURBIDITY CURRENTS: PENNSYLVANIAN MINTURN FORMATION, COLORADO, U.S.A. 1 2 2 3 2 M. P. LAMB, P. M. MYROW, C. LUKENS, K. HOUCK, AND J. STRAUSS 1Department of Earth & Planetary Science, University of California, Berkeley, California 94720, U.S.A. 2Department of Geology, Colorado College, Colorado Springs, Colorado 80903, U.S.A. 3Department of Geography and Environmental Sciences, University of Colorado, Denver, Colorado, 80217-3363 U.S.A. e-mail: [email protected] ABSTRACT: Turbidity currents generated nearshore have been suggested to be the source of some sandy marine event beds, but in most cases the evidence is circumstantial. Such flows must commonly travel through a field of oscillatory flow caused by wind-generated waves; little is known, however, about the interactions between waves and turbidity currents. We explore these interactions through detailed process-oriented sedimentological analysis of sandstone event beds from the Pennsylvanian Minturn Formation in north-central Colorado, U.S.A. The Minturn Formation exhibits a complex stratigraphic architecture of fan-delta deposits that developed in association with high topographic relief in a tectonically active setting. An , 20–35-m- thick, unconformity-bounded unit of prodelta deposits consists of dark green shale and turbidite-like sandstone beds with tool marks produced by abundant plant debris. Some of the sandstone event beds, most abundant at distal localities, contain reverse- to-normal grading and sequences of sedimentary structures that indicate deposition from waxing to waning flows. In contrast, proximal deposits, in some cases less than a kilometer away, contain abundant beds with evidence for deposition by wave- dominated combined flows, including large-scale hummocky cross-stratification (HCS). -
1 Running Head: SEQUENCE STRATIGRAPHY of TEXAS
Running Head: SEQUENCE STRATIGRAPHY OF TEXAS MIDDLE PERMIAN PLATFORM CARBONATES OUTCROP-BASED CHARACTERiZATION OF LEONARDIAN PLATFORM CARBONATE IN WEST TEXAS: IMPLICATIONS FOR SEQUENCE STRATIGRAPHIC STYLES IN TRANSITIONAL ICEHOUSE-GREENHOUSE SETTINGS Stephen C. Ruppel, W. Bruce Ward1, and Eduardo E. Ariza Bureau of Economic Geology The University of Texas at Austin 1 Current address: Earthworks LLC, P.O. Box 178, Newtown, CT 06470-0178 1 ABSTRACT The Sierra Diablo Mountains of West Texas contain world class exposures of lower and middle Permian platform carbonates. As such these outcrops offer key insights into the products of carbonate deposition in the transitional icehouse/greenhouse setting of the early-mid Permian that are available in few other places in the world. They also afford an excellent basis for examing how styles of facies and sequence development vary between platform tops and platform margins. Using outcrop data and observations from over 2 mi (3 km) of continuous exposure, we collected detailed data on the facies composition and architecture of high frequency (cycle-scale) and intermediate frequency (high frequency sequence scale) successions within the Leonardian. We used these data to define facies stacking patterns along depositional dip across the platform in both low and high accommodation settings and to document how these patterns vary systematically between and within sequences . These data not only provide a basis for interpreting similar Leonardian platform successions from less well constrained outcrop and subsurface data sets but also point out some important caveats that should be considered serve as an important model for understanding depositional processes during the is part of the Permian worldwide. -
(LOWER PENNSYLVANIAN) in the PERMIAN BASIN Wayne R. Wright Bureau of Economic Geol
DEPOSITIONAL HISTORY OF THE ATOKAN SUCCESSION (LOWER PENNSYLVANIAN) IN THE PERMIAN BASIN Wayne R. Wright Bureau of Economic Geology Jackson School of Geosciences The University of Texas at Austin Austin, Texas ABSTRACT Atokan-age units in the Permian Basin record a 2nd-order transgression, with aerially restricted, lower Atokan fluvial to shallow-marine siliciclastics followed by pervasive carbonate deposition. In general, Atokan-age siliciclastics dominated deposition in the west of the Permian Basin while carbonate deposition dominated throughout the rest of the basin. Predominance of carbonate facies across most of the Permian Basin is due to (1) lack of siliciclastic supply, (2) overall 2nd-order rising sea level, and (3) progradation of the Upper Marble Falls Formation onto the Eastern Shelf. Progradation was due partly to lower accommodation to the west and backstepping/retreat from encroaching Atokan deltaics to the east. The beginning of the Atokan is marked by a sea-level drop and subsequent lowstand conditions. A sequence boundary separates the Atokan from the underlying Morrowan carbonate section throughout the Permian Basin. Siliciclastic deposition in and around the Permian Basin is more aerially restricted than in the Morrowan. The earliest Atokan lowstand event is manifested in alluvial and fluvial incised-valley sediments in Lea County, New Mexico, and the Broken Bone Graben (Cottle County, Texas); fan- delta deposits in the Palo Duro Basin and Taylor Draw field (Upton County, Texas); and 1 post-Lower Marble Falls–pre-Upper Marble falls conglomerates (Gibbons Formation?) on the Llano Uplift. Following the lowstand event, a 2nd-order transgression appears to have dominated throughout the rest of the Atokan; however 3rd- and 4th-order, high- amplitude, sea-level fluctuations also occurred. -
Permian Basin, West Texas and Southeastern New Mexico
Report of Investigations No. 201 Stratigraphic Analysis of the Upper Devonian Woodford Formation, Permian Basin, West Texas and Southeastern New Mexico John B. Comer* *Current address Indiana Geological Survey Bloomington, Indiana 47405 1991 Bureau of Economic Geology • W. L. Fisher, Director The University of Texas at Austin • Austin, Texas 78713-7508 Contents Abstract ..............................................................................................................................1 Introduction ..................................................................................................................... 1 Methods .............................................................................................................................3 Stratigraphy .....................................................................................................................5 Nomenclature ...................................................................................................................5 Age and Correlation ........................................................................................................6 Previous Work .................................................................................................................6 Western Outcrop Belt ......................................................................................................6 Central Texas ...................................................................................................................7 Northeastern Oklahoma -
Depositional Setting and Reservoir-Scale
DEPOSITIONAL SETTING AND RESERVOIR-SCALE ARCHITECTURE OF SANDSTONE BODIES OF THE GREEN RIVER FORMATION IN EVACUATION CREEK, DRAGON QUADRANGLE, EASTERN UINTA BASIN, UTAH by T. Ryan O’Hara A thesis submitted to the Faculty and the Board of Trustees of the Colorado School of Mines in partial fulfillment of the requirements for the degree of Master of Science (Geology) Golden, Colorado Date _____________ Signed: ________________________________ T. Ryan O’Hara Signed: ________________________________ Dr. J. Frederick Sarg Thesis Advisor Golden, Colorado Date _____________ Signed: ________________________________ Dr. Paul Santi Professor and Head Department of Geology and Geologic Engineering ii ABSTRACT The Green River Formation is an Eocene lacustrine deposit that is present in several Rocky Mountain basins. In the Uinta basin, the Green River Formation has produced large amounts of oil and gas from many fields, the largest being the Greater Altamont-Bluebell field in the northern margin of the basin, the Monument Butte and Natural Buttes fields in the central region, and the Greater Red Wash field in the northeastern part of the basin. In addition, the Green River Formation contains one of the largest oil shale deposits in the world. The Uinta and Piceance basins are estimated to contain 1.32 trillion barrels and 1.53 trillion barrels respectively of total in-place oil shale resource. This study focuses on littoral to sublittoral sandstone deposition of the Green River Formation in the eastern Uinta basin of Utah, in Evacuation Creek, near the Utah-Colorado border. This area contains extensive and continuous outcrop of the Green River Formation exposed in steep cliffs and gullies, and allows for the study of these units at the reservoir-scale. -
U.S. Geological Survey Bulletin 1839-G, H
Stratigraphic Framework of Cambrian and Ordovician Rocks in the Central Appalachian Basin from Morrow County, Ohio, to Pendleton County, West Virginia Depositional Environment of the Fincastle Conglomerate near Roanoke, Virginia U.S. GEOLOGICAL SURVEY BULLETIN 1839-G, H i i i I ' i ' i ' X- »-v l^,:^ Stratigraphic Framework of Cambrian and Ordovician Rocks in the Central Appalachian Basin from Morrow County, Ohio, to Pendleton County, West Virginia By ROBERT T. RYDER Depositional Environment of the Fincastle Conglomerate near Roanoke, Virginia By CHRYSA M. CULLATHER Chapters G and H are issued as a single volume and are not available separately U.S. GEOLOGICAL SURVEY BULLETIN 1839-G, H EVOLUTION OF SEDIMENTARY BASINS-APPALACHIAN BASIN U.S. DEPARTMENT OF THE INTERIOR MANUEL LUJAN, Jr., Secretary U.S. GEOLOGICAL SURVEY DALLAS L. PECK, Director Any use of trade, product, or firm names in this publication is for descriptive purposes only and does not imply endorsement by the U.S. Government UNITED STATES GOVERNMENT PRINTING OFFICE: 1992 For sale by Book and Open-File Report Sales U.S. Geological Survey Federal Center, Box 25425 Denver, CO 80225 Library of Congress Cataloging in Publication Data (revised for vol. G-H) Evoluation of sedimentary basins Appalachian basin. (U.S. Geological Survey bulletin ; 1839 A-D, G-H) Includes bibliographies. Supt. of Docs. no.:19.3:1839-G Contents: Horses in fensters of the Pulaski thrust sheet, southwestern Virginia / by Arthur P. Schultz [etc.] Stratigraphic framework of Cam brian and Ordovician rocks in central Appalachian basin from Morrow County, Ohio, to Pendleton County, West Virginia / by Robert T. -
Geological Survey Standards. CONTENTS
UNITED STATES DEPARTMENT OF THE INTERIOR GEOLOGICAL SURVEY Depositional Environment of the Middle Proterozoic Spokane Formation-Empire Formation Transition Zone, West-Central Montana By James W. Whipple Open-File Report 80-1232 1980 This report is preliminary and has not been edited or reviewed for conformity with U.S. Geological Survey standards. CONTENTS Page Introduction*........................................................... 1 Acknowledgments.................................................... A Purpose and scope.................................................. A Previous studies................................................... A Geologic setting................................................... 5 Stratigraphic subdivision.......................................... 6 Primary sedimentary structures.......................................... 7 Bedding and lamination............................................. 8 Graded lamination............................................. 8 Planar lamination............................................. 11 Rhythmic sand and mud bedding................................. 1A Wavy lamination............................................... 1A Ripple bedding and lamination................................. 17 Algal lamination and loferites................................ 19 Bedding-plane marks and imprints................................... 19 Subaerial shrinkage cracks.................................... 21 Subaqueous shrinkage cracks................................... 21 Raindrop impressions......................................... -
Bulletin of the Geological Society of Denmark, Vol. 35/1-2, Pp. 19-23
Soft sediment deformation structures in Silurian turbidites from North Greenland POUL-HENRIK LARSEN Larsen, P.-H.: Soft sediment deformation structures in Silurian turbidites from North Greenland. Bull, DGF geol. Soc. Denmark, vol. 35, pp. 19-23, Copenhagen, October, 29th, 1986. Turbidite beds in the Silurian turbidite sequence, North Greenland, show soft sediment deformation struc tures suggesting that the structureless (in respect of traction structures) sandstones divisions of the turbidi tes were deposited by direct suspension sedimentation from high-density flows. The deposits may have re sulted from multiple successive depositional events within the same turbidity flow. Reworking and shear ing of the newly formed loosely packed high-density suspension deposits caused by the still moving flow above create secondary soft sediment deformation structures which may be used as current indicators if other structures are absent (e.g. flute casts). P.-H. Larsen, Grønlands Geologiske Undersøgelser, Øster Voldgade 10, DK-1350 Copenhagen K, Den mark. May 6th, I985. General aspects of turbidite sedimentation fine sand, silt and clay in a turbulent suspension. These residual currents may complete bypass The organisation of deposits from low-density areas of high-density turbidity current deposi turbidity currents, the classical Bouma model, tion, but may have significant local effects. They has been extensively discussed and reviewed by may shear, liquify and homogenise the loosely Bouma (1962), Walker (1965), Sanders (1965), packed high-density suspension deposits (Mid Middleton (1967, 1969, 1970), Walton (1967), dleton 1967; Lowe 1982). Unsteady, residual low Allen (1970) and Middleton & Hampton (1973, density currents can deposit sediment above that 1976) among others. -
A New Late Permian Burnetiamorph from Zambia Confirms Exceptional
fevo-09-685244 June 19, 2021 Time: 17:19 # 1 ORIGINAL RESEARCH published: 24 June 2021 doi: 10.3389/fevo.2021.685244 A New Late Permian Burnetiamorph From Zambia Confirms Exceptional Levels of Endemism in Burnetiamorpha (Therapsida: Biarmosuchia) and an Updated Paleoenvironmental Interpretation of the Upper Madumabisa Mudstone Formation Edited by: 1 † 2 3,4† Mark Joseph MacDougall, Christian A. Sidor * , Neil J. Tabor and Roger M. H. Smith Museum of Natural History Berlin 1 Burke Museum and Department of Biology, University of Washington, Seattle, WA, United States, 2 Roy M. Huffington (MfN), Germany Department of Earth Sciences, Southern Methodist University, Dallas, TX, United States, 3 Evolutionary Studies Institute, Reviewed by: University of the Witwatersrand, Johannesburg, South Africa, 4 Iziko South African Museum, Cape Town, South Africa Sean P. Modesto, Cape Breton University, Canada Michael Oliver Day, A new burnetiamorph therapsid, Isengops luangwensis, gen. et sp. nov., is described Natural History Museum, on the basis of a partial skull from the upper Madumabisa Mudstone Formation of the United Kingdom Luangwa Basin of northeastern Zambia. Isengops is diagnosed by reduced palatal *Correspondence: Christian A. Sidor dentition, a ridge-like palatine-pterygoid boss, a palatal exposure of the jugal that [email protected] extends far anteriorly, a tall trigonal pyramid-shaped supraorbital boss, and a recess †ORCID: along the dorsal margin of the lateral temporal fenestra. The upper Madumabisa Christian A. Sidor Mudstone Formation was deposited in a rift basin with lithofacies characterized orcid.org/0000-0003-0742-4829 Roger M. H. Smith by unchannelized flow, periods of subaerial desiccation and non-deposition, and orcid.org/0000-0001-6806-1983 pedogenesis, and can be biostratigraphically tied to the upper Cistecephalus Assemblage Zone of South Africa, suggesting a Wuchiapingian age. -
Stratigraphy and Sedimentology of a Dry to Wet Eolian Depositional System, Burns Formation, Meridiani Planum, Mars
Earth and Planetary Science Letters 240 (2005) 11–72 www.elsevier.com/locate/epsl Stratigraphy and sedimentology of a dry to wet eolian depositional system, Burns formation, Meridiani Planum, Mars J.P. Grotzinger a,*, R.E. Arvidson b, J.F. Bell III c, W. Calvin d, B.C. Clark e, D.A. Fike a, M. Golombek f, R. Greeley g, A. Haldemann f, K.E. Herkenhoff h, B.L. Jolliff b, A.H. Knoll i, M. Malin j, S.M. McLennan k, T. Parker e, L. Soderblom g, J.N. Sohl-Dickstein b, S.W. Squyres b, N.J. Tosca k, W.A. Watters a a Massachusetts Inst. of Technology, Earth, Atmos. and Planetary Sci., Cambridge, MA 02139, USA b Department Earth and Planetary Sciences, Washington University, St. Louis, MO 63130, USA c Department of Astronomy, Space Sciences Bldg. Cornell University, Ithaca, NY 14853, USA d University of Nevada, Reno, NV 89501, USA e Lockheed Martin Corporation, Littleton, CO 80127, USA f Jet Propulsion Laboratory, California Institute of Technology, Pasadena, CA 91109, USA g Department Geological Sciences, Arizona State University, Box 871404, Tempe, AZ 85287-1404, USA h U.S. Geological Survey, Flagstaff, AZ 86001, USA i Botanical Museum, Harvard University, Cambridge MA 02138, USA j Malin Space Science Systems, Inc., San Diego, CA 92191, USA k Department of Geosciences, State University of New York, Stony Brook, NY 11794-2100, USA Accepted 22 September 2005 Editor: A.N. Halliday Abstract Outcrop exposures of sedimentary rocks at the Opportunity landing site (Meridiani Planum) form a set of genetically related strata defined here informally as the Burns formation. -
Play Analysis of Major Oil Reservoirs in the New Mexico Part of the Permian Basin: Enhanced Production Through Advanced Technologies by Ronald F
Play Analysis of Major Oil Reservoirs in the New Mexico Part of the Permian Basin: Enhanced Production Through Advanced Technologies by 1 2 3 Ronald F. Broadhead , Zhou Jianhua and William D. Raatz 1New Mexico Bureau of Geology and Mineral Resources, a division of New Mexico Tech, Socorro NM 87801 2Department of Computer Sciences, New Mexico Tech, Socorro NM 87801 3New Mexico Bureau of Geology and Mineral Resources, present address OxyPermian, Houston, TX From R.L. Martin and K.F. Hickey West Texas Geological Society, 2002 Open File Report 479 New Mexico Bureau of Geology and Mineral Resources, A division of New Mexico Tech Socorro, NM 87801 Peter A. Scholle, Director July 2004 DISCLAIMER This open-file report was prepared with the support of the U.S. Department of Energy, under Award No. DE-FC26-02NT15131. However, any opinions, findings, conclusions, or recommendations expressed herein are those of the authors and do not necessarily reflect the views of the DOE. This report was prepared as an account of work sponsored by an agency of the United States Government. Neither the United States Government nor any agency thereof, nor any of their employees, makes any warranty, express or implicit, or assumes any legal liability for the responsibility for the accuracy, completeness, or usefulness of any information, apparatus, product, or process disclosed, or represents that its use would not infringe privately owned rights. Reference herein to any specific commercial product, process, or service by trade name, trademark, manufacturer, or otherwise does not necessarily constitute or imply its endorsement, recommendation, or favoring by the United States Government or any agency thereof.