Ocellar Spatial Vision in Myrmecia Ants
Total Page:16
File Type:pdf, Size:1020Kb
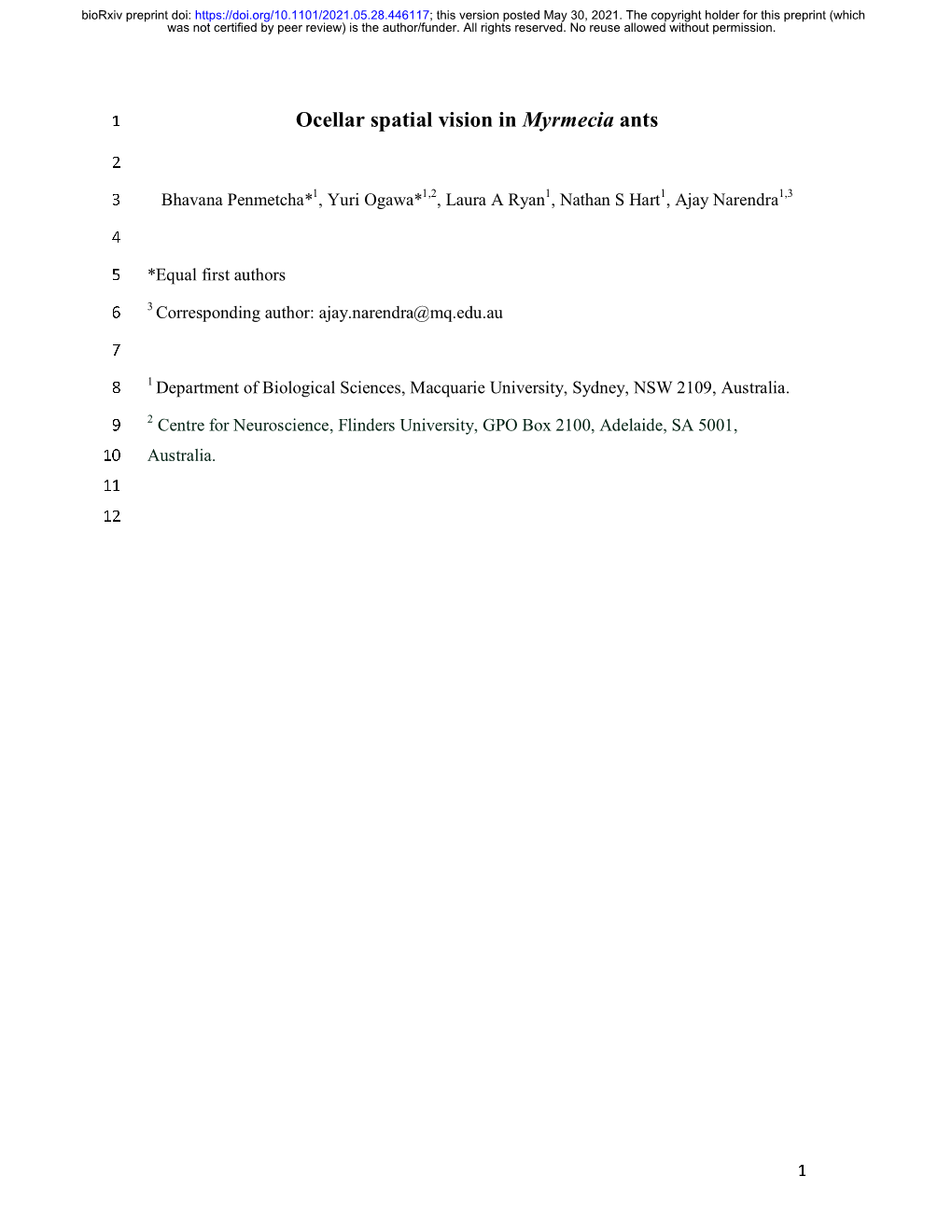
Load more
Recommended publications
-
Observations on Forced Colony Emigration in Parachartergus Fraternus (Hymenoptera: Vespidae: Epiponini): New Nest Site Marked with Sprayed Venom
Hindawi Publishing Corporation Psyche Volume 2011, Article ID 157149, 8 pages doi:10.1155/2011/157149 Research Article Observations on Forced Colony Emigration in Parachartergus fraternus (Hymenoptera: Vespidae: Epiponini): New Nest Site Marked with Sprayed Venom Sidnei Mateus Departamento de Biologia, Faculdade de Filosofia CiˆenciaseLetrasdeRibeir˜ao Preto, Universidade de S˜ao Paulo, Avenida Bandeirantes 3900, 14040-901 Ribeir˜ao Preto, SP, Brazil Correspondence should be addressed to Sidnei Mateus, sidneim@ffclrp.usp.br Received 8 September 2010; Revised 20 December 2010; Accepted 12 February 2011 Academic Editor: Robert Matthews Copyright © 2011 Sidnei Mateus. This is an open access article distributed under the Creative Commons Attribution License, which permits unrestricted use, distribution, and reproduction in any medium, provided the original work is properly cited. Five cases of colony emigration induced by removal of nest envelope and combs and a single one by manipulation are described. The disturbance was followed by defensive patterns, buzz running, and adult dispersion. An odor trail created by abdomen dragging, probably depositing venom or Dufour’s gland secretions, connected the original nest to the newly selected nesting place and guided the emigration. The substrate of the selected nesting place is intensely sprayed with venom prior to emigration, and this chemical cue marked the emigration end point. The colony moves to the new site in a diffuse cloud with no temporary clusters formed along the odor trail. At the original nest, scouts performed rapid gaster dragging and intense mouth contacts stimulating inactive individuals to depart. Males were unable to follow the swarm. Individual scouts switched between different behavioral tasks before and after colony emigration. -
Visual Ecology of Indian Carpenter Bees II: Adaptations of Eyes and Ocelli to Nocturnal and Diurnal Lifestyles
J Comp Physiol A (2009) 195:571–583 DOI 10.1007/s00359-009-0432-9 ORIGINAL PAPER Visual ecology of Indian carpenter bees II: adaptations of eyes and ocelli to nocturnal and diurnal lifestyles Hema Somanathan Æ Almut Kelber Æ Renee M. Borges Æ Rita Walle´n Æ Eric J. Warrant Received: 11 December 2008 / Revised: 25 February 2009 / Accepted: 4 March 2009 / Published online: 11 April 2009 Ó Springer-Verlag 2009 Abstract Most bees are diurnal, with behaviour that is Keywords Apposition compound eyes Á Bees Á largely visually mediated, but several groups have made Ocelli Á Optical sensitivity Á Xylocopa evolutionary shifts to nocturnality, despite having apposi- tion compound eyes unsuited to vision in dim light. We compared the anatomy and optics of the apposition eyes Introduction and the ocelli of the nocturnal carpenter bee, Xylocopa tranquebarica, with two sympatric species, the strictly The apposition compound eye is the predominant eye diurnal X. leucothorax and the occasionally crepuscular X. design in most diurnal insects including bees (Land and tenuiscapa. The ocelli of the nocturnal X. tranquebarica Nilsson 2002). In these eyes, each visual unit, or omma- are unusually large (diameter ca. 1 mm) and poorly tidium, consists of a corneal lens, a crystalline cone and focussed. Moreover, their apposition eyes show specific photoreceptor cells surrounded by screening pigment that visual adaptations for vision in dim light, including large absorbs incoming off-axis light. The rhabdom, the light- size, large facets and very wide rhabdoms, which together sensitive portion of the photoreceptor cells, receives only make these eyes 9 times more sensitive than those of X. -
Vertical Lobes of the Mushroom Bodies Are Essential for View
Please cite this article in press as: Kamhi et al., Vertical Lobes of the Mushroom Bodies Are Essential for View-Based Navigation in Australian Myrmecia Ants, Current Biology (2020), https://doi.org/10.1016/j.cub.2020.06.030 ll Report Vertical Lobes of the Mushroom Bodies Are Essential for View-Based Navigation in Australian Myrmecia Ants J. Frances Kamhi,1,2 Andrew B. Barron,1 and Ajay Narendra1,3,* 1Department of Biological Sciences, Macquarie University, Sydney, NSW 2109, Australia 2Neuroscience Department, Oberlin College, Oberlin, OH 44074, USA 3Lead Contact *Correspondence: [email protected] https://doi.org/10.1016/j.cub.2020.06.030 SUMMARY Prior to leaving home, insects acquire visual landmark information through a series of well-choreographed walks or flights of learning [1–4]. This information allows them to pinpoint goals both when in their vicinity [5–7] and from locations they have not previously visited [8–10]. It is presumed that animals returning home match memorized views to their current view for successful view-based navigation [11]. While view- based navigation strategies have been incorporated into several navigation models [8, 12, 13], we still know little about how this behavior is performed by the insect brain. Mushroom bodies are essential for visual learning and memory [14–16], and therefore we investigated their role in view-based navigation in a visually oriented ant, Myrmecia midas. We injected the local anesthetic procaine [15, 17, 18] into the mushroom body vertical lobes (VLs) to selectively inhibit neural activity in this region. We compared the behavior of VL-pro- caine-treated ants with three groups: untreated control, VL-saline, and off-target (antennal lobe) procaine. -
Hymenoptera: Vespidae; Polistinae, Epiponini)
PUBLISHED BY THE AMERICAN MUSEUM OF NATURAL HISTORY CENTRAL PARK WEST AT 79TH STREET, NEW YORK, NY 10024 Number 3562, 30 pp., 22 figures, 1 table May 16, 2007 Revision and Cladistic Analysis of the Nocturnal Social Wasp Genus, Apoica Lepeletier (Hymenoptera: Vespidae; Polistinae, Epiponini) KURT M. PICKETT1,3 AND JOHN W. WENZEL2 ABSTRACT A revision of the nocturnal social wasp genus, Apoica, is presented. The revision is based on a cladistic analysis of morphological, behavioral, and molecular characters of the nine nominal species. The subgenera Deuterapoica and Apoica are consistent with the phylogenetic results, and are thus retained. A new species, Apoica ellenae, whose phylogenetic position is uncertain, is described. A variant of A. albimacula from Peru is noted. This brings the total number of species in the genus to 10. INTRODUCTION also unusual. In some cases, queens appear to be slightly smaller than workers (Richards, The paper wasp genus Apoica Lepeletier is 1978), which runs counter to the typical one of the most bizarre genera of the swarm- situation. Other studies have demonstrated founding Polistinae, or tribe Epiponini. Its that queens and workers are approximately nocturnal habit is unique within the the same size (though queens are larger than Epiponini, and with the exception of the workers posteriorly, and workers larger than vespine Provespa (see review in Matsuura, queens anteriorly [Jeanne et al., 1995; Noll et 1991), no other social wasp genus is primarily al., 2004]), and this too seems to preclude the nocturnal. Linked to its nocturnal habit, typical mode of caste determination, in which Apoica is characterized by unusually large morphological caste differences are due to ocelli. -
It Takes Two: Dimerization Is Essential for the Broad-Spectrum Predatory and Defensive Activities of the Venom Peptide Mp1a from the Jack Jumper Ant Myrmecia Pilosula
biomedicines Article It Takes Two: Dimerization Is Essential for the Broad-Spectrum Predatory and Defensive Activities of the Venom Peptide Mp1a from the Jack Jumper Ant Myrmecia pilosula Samantha A. Nixon 1,2 , Zoltan Dekan 1 , Samuel D. Robinson 1, Shaodong Guo 1 , Irina Vetter 1,3 , Andrew C. Kotze 2, Paul F. Alewood 1, Glenn F. King 1,* and Volker Herzig 1,4,* 1 Institute for Molecular Bioscience, The University of Queensland, St Lucia, QLD 4072, Australia; [email protected] (S.A.N.); [email protected] (Z.D.); [email protected] (S.D.R.); [email protected] (S.G.); [email protected] (I.V.); [email protected] (P.F.A.) 2 CSIRO Agriculture and Food, St Lucia, QLD 4072, Australia; [email protected] 3 School of Pharmacy, The University of Queensland, Woolloongabba, QLD 4102, Australia 4 School of Science & Engineering, University of the Sunshine Coast, Sippy Downs, QLD 4556, Australia * Correspondence: [email protected] (G.F.K.); [email protected] (V.H.); Tel.: +61-7-3346-2025 (G.F.K.); +61-7-5456-5382 (V.H.) Received: 11 June 2020; Accepted: 24 June 2020; Published: 30 June 2020 Abstract: Ant venoms have recently attracted increased attention due to their chemical complexity, novel molecular frameworks, and diverse biological activities. The heterodimeric peptide D-myrtoxin-Mp1a (Mp1a) from the venom of the Australian jack jumper ant, Myrmecia pilosula, exhibits antimicrobial, membrane-disrupting, and pain-inducing activities. In the present study, we examined the activity of Mp1a and a panel of synthetic analogues against the gastrointestinal parasitic nematode Haemonchus contortus, the fruit fly Drosophila melanogaster, and for their ability to stimulate pain-sensing neurons. -
Vespas Sociais (Vespidae: Polistinae)
doi:10.12741/ebrasilis.v7i3.404 e-ISSN 1983-0572 Publicação do Projeto Entomologistas do Brasil www.ebras.bio.br Distribuído através da Creative Commons Licence v3.0 (BY-NC-ND) Copyright © EntomoBrasilis Copyright © do(s) Autor(es) Vespas Sociais (Vespidae: Polistinae) em uma Área de Floresta Ombrófila Densa Amazônica no Estado do Maranhão, Brasil Alexandre Somavilla¹, Dayse Willkenia Almeida Marques¹, Ernesto Augusto Silva Barbosa¹, Juarez da Silva Pinto Junior² & Marcio Luiz de Oliveira¹ 1. Instituto de Pesquisas da Amazônia, Programa de Pós-Graduação em Entomologia, e-mail: [email protected] (Autor para correspondência), [email protected], [email protected], [email protected]. 2. Universidade Estadual do Maranhão, e-mail: [email protected]. _____________________________________ EntomoBrasilis 7 (3): 183-187 (2014) Resumo. As vespas sociais constituem um grupo com elevada riqueza de espécies e muito comum em áreas amazônicas. Apesar disto, nenhum trabalho foi realizado na Amazônia Maranhense e apenas 58 espécies de vespas sociais são registradas para o estado do Maranhão. O presente trabalho apresenta dados faunísticos referentes às vespas sociais da Reserva Biológica do Gurupi, caracterizada por ser uma floresta ombrófila latifoliada amazônica localizada no noroeste do Maranhão. As coletas e instalação de armadilhas (Malaise do tipo Gressitt & Gressitt, armadilha suspensa, armadilha luminosa, armadilha atrativa com isca de fruta) ocorreram entre janeiro de 2010 e maio de 2011, totalizando 63 dias amostrados. Para a Reserva Biológica do Gurupi foram obtidos 384 espécimes de vespas sociais pertencentes a Epiponini e Polistini, alocados em 12 gêneros e 38 espécies. Polybia destacou-se por apresentar o maior número de espécies (14), seguido de Agelaia (seis espécies) e Apoica (quatro espécies). -
Picture As Pdf Download
RESEARCH Causes of ant sting anaphylaxis in Australia: the Australian Ant Venom Allergy Study Simon G A Brown, Pauline van Eeden, Michael D Wiese, Raymond J Mullins, Graham O Solley, Robert Puy, Robert W Taylor and Robert J Heddle he prevalence of systemic allergy to ABSTRACT native ant stings in Australia is as high as 3% in areas where these Objective: To determine the Australian native ant species associated with ant sting T anaphylaxis, geographical distribution of allergic reactions, and feasibility of diagnostic insects are commonly encountered, such as Tasmania and regional Victoria.1,2 In one venom-specific IgE (sIgE) testing. large Tasmanian emergency department Design, setting and participants: Descriptive clinical, entomological and study, ant sting allergy was the most com- immunological study of Australians with a history of ant sting anaphylaxis, recruited in mon cause of anaphylaxis (30%), exceeding 2006–2007 through media exposure and referrals from allergy practices and emergency cases attributed to bees, wasps, antibiotics physicians nationwide. We interviewed participants, collected entomological or food.3 specimens, prepared reference venom extracts, and conducted serum sIgE testing Myrmecia pilosula (jack jumper ant [JJA]) against ant venom panels relevant to the species found in each geographical region. is theThe major Medical cause Journal of ant ofsting Australia anaphylaxis ISSN: Main outcome measures: Reaction causation attributed using a combination of ant 2 in Tasmania.0025-729X A 18double-blind, July 2011 195 randomised 2 69-73 identification and sIgE testing. placebo-controlled©The Medical Journaltrial has of Australiademonstrated 2011 Results: 376 participants reported 735 systemic reactions. Of 299 participants for whom the effectivenesswww.mja.com.au of JJA venom immuno- a cause was determined, 265 (89%; 95% CI, 84%–92%) had reacted clinically to Myrmecia therapyResearch (VIT) to reduce the risk of sting species and 34 (11%; 95% CI, 8%–16%) to green-head ant (Rhytidoponera metallica). -
Differential Investment in Brain Regions for a Diurnal and Nocturnal Lifestyle in Australian Myrmecia Ants
Received: 12 July 2018 Revised: 7 December 2018 Accepted: 22 December 2018 DOI: 10.1002/cne.24617 RESEARCH ARTICLE Differential investment in brain regions for a diurnal and nocturnal lifestyle in Australian Myrmecia ants Zachary B. V. Sheehan1 | J. Frances Kamhi1 | Marc A. Seid1,2 | Ajay Narendra1 1Department of Biological Sciences, Macquarie University, Sydney, New South Wales, Abstract Australia Animals are active at different times of the day. Each temporal niche offers a unique light envi- 2Biology Department, Neuroscience Program, ronment, which affects the quality of the available visual information. To access reliable visual The University of Scranton, Scranton, signals in dim-light environments, insects have evolved several visual adaptations to enhance Pennsylvania their optical sensitivity. The extent to which these adaptations reflect on the sensory processing Correspondence Department of Biological Sciences, Macquarie and integration capabilities within the brain of a nocturnal insect is unknown. To address this, University, 205 Culloden Road, Sydney, NSW we analyzed brain organization in congeneric species of the Australian bull ant, Myrmecia, that 2109, Australia. rely predominantly on visual information and range from being strictly diurnal to strictly noctur- Email: [email protected] nal. Weighing brains and optic lobes of seven Myrmecia species, showed that after controlling Funding information for body mass, the brain mass was not significantly different between diurnal and nocturnal Australian Research Council, Grant/Award Numbers: DP150101172, FT140100221 ants. However, the optic lobe mass, after controlling for central brain mass, differed between day- and night-active ants. Detailed volumetric analyses showed that the nocturnal ants invested relatively less in the primary visual processing regions but relatively more in both the primary olfactory processing regions and in the integration centers of visual and olfactory sen- sory information. -
Compass Cues Used by a Nocturnal Bull Ant, Myrmecia Midas Cody A
© 2017. Published by The Company of Biologists Ltd | Journal of Experimental Biology (2017) 220, 1578-1585 doi:10.1242/jeb.152967 RESEARCH ARTICLE Compass cues used by a nocturnal bull ant, Myrmecia midas Cody A. Freas*, Ajay Narendra and Ken Cheng ABSTRACT skylight derived from the sun (e.g. Zeil et al., 2014b). The Ants use both terrestrial landmarks and celestial cues to navigate to polarization information is acquired through a specialized dorsal ’ and from their nest location. These cues persist even as light levels region of the ant s eyes (e.g. Zeil et al., 2014b; Narendra et al., drop during the twilight/night. Here, we determined the compass cues 2016b) and is processed via polarization-sensitive optic lobe used by a nocturnal bull ant, Myrmecia midas, in which the majority of neurons (Schmitt et al., 2015). This directional information is individuals begin foraging during the evening twilight period. coupled with distance information, which the ant accumulates as it Myrmecia midas foragers with vectors of ≤5 m when displaced to travels away from the nest. To return home, ants integrate these two unfamiliar locations did not follow the home vector, but instead sources of information and compute the shortest home vector (e.g. showed random heading directions. Foragers with larger home Collett and Collett, 2000; Wehner and Srinivasan, 2003). vectors (≥10 m) oriented towards the fictive nest, indicating a possible These vision-based navigational abilities have been widely increase in cue strength with vector length. When the ants were studied in diurnal ants, which are active when visual cues are easy displaced locally to create a conflict between the home direction to distinguish (Wehner et al., 1996; Fukushi, 2001; Beugnon et al., indicated by the path integrator and terrestrial landmarks, foragers 2005; Cheng et al., 2009; Bühlmann et al., 2011). -
Comparative Morphology of the Stinger in Social Wasps (Hymenoptera: Vespidae)
insects Article Comparative Morphology of the Stinger in Social Wasps (Hymenoptera: Vespidae) Mario Bissessarsingh 1,2 and Christopher K. Starr 1,* 1 Department of Life Sciences, University of the West Indies, St Augustine, Trinidad and Tobago; [email protected] 2 San Fernando East Secondary School, Pleasantville, Trinidad and Tobago * Correspondence: [email protected] Simple Summary: Both solitary and social wasps have a fully functional venom apparatus and can deliver painful stings, which they do in self-defense. However, solitary wasps sting in subduing prey, while social wasps do so in defense of the colony. The structure of the stinger is remarkably uniform across the large family that comprises both solitary and social species. The most notable source of variation is in the number and strength of barbs at the tips of the slender sting lancets that penetrate the wound in stinging. These are more numerous and robust in New World social species with very large colonies, so that in stinging human skin they often cannot be withdrawn, leading to sting autotomy, which is fatal to the wasp. This phenomenon is well-known from honey bees. Abstract: The physical features of the stinger are compared in 51 species of vespid wasps: 4 eumenines and zethines, 2 stenogastrines, 16 independent-founding polistines, 13 swarm-founding New World polistines, and 16 vespines. The overall structure of the stinger is remarkably uniform within the family. Although the wasps show a broad range in body size and social habits, the central part of Citation: Bissessarsingh, M.; Starr, the venom-delivery apparatus—the sting shaft—varies only to a modest extent in length relative to C.K. -
Detour Learning Ability and the Effect of Novel Sensory Cues On
bioRxiv preprint doi: https://doi.org/10.1101/2021.01.25.428158; this version posted January 27, 2021. The copyright holder for this preprint (which was not certified by peer review) is the author/funder. All rights reserved. No reuse allowed without permission. 1 Detour learning ability and the effect of novel sensory cues on 2 learning in Australian bull ants, Myrmecia midas 3 4 Muzahid Islam1, Sudhakar Deeti1, Zakia Mahmudah1, J. Frances Kamhi1,2, Ken Cheng1 5 6 1Department of Biological Sciences, Macquarie University, Sydney, Australia 7 2Neuroscience Department, Oberlin College, Oberlin, Ohio, USA 8 9 Subject Category: Neuroscience and Cognition 10 Running Head: Learning & Cognitive ability of bull ant 11 12 Keywords: Detour Learning, Successful Foragers, Path Straightness, Motor Routine 13 14 Author for correspondence: 15 Muzahid Islam 16 e-mail: [email protected] 17 bioRxiv preprint doi: https://doi.org/10.1101/2021.01.25.428158; this version posted January 27, 2021. The copyright holder for this preprint (which was not certified by peer review) is the author/funder. All rights reserved. No reuse allowed without permission. 18 Detour learning ability and the effect of novel sensory cues on 19 learning in Australian bull ants, Myrmecia midas 20 21 ABSTRACT 22 Many animals navigate in a structurally complex environment which requires them to detour around 23 physical barriers that they encounter. While many studies in animal cognition suggest that they are able to 24 adeptly avoid obstacles, it is unclear whether a new route is learned to navigate around these barriers and, 25 if so, what sensory information may be used to do so. -
Biodiversity Summary for NRM Regions Species List
Biodiversity Summary for NRM Regions Species List What is the summary for and where does it come from? This list has been produced by the Department of Sustainability, Environment, Water, Population and Communities (SEWPC) for the Natural Resource Management Spatial Information System. The list was produced using the AustralianAustralian Natural Natural Heritage Heritage Assessment Assessment Tool Tool (ANHAT), which analyses data from a range of plant and animal surveys and collections from across Australia to automatically generate a report for each NRM region. Data sources (Appendix 2) include national and state herbaria, museums, state governments, CSIRO, Birds Australia and a range of surveys conducted by or for DEWHA. For each family of plant and animal covered by ANHAT (Appendix 1), this document gives the number of species in the country and how many of them are found in the region. It also identifies species listed as Vulnerable, Critically Endangered, Endangered or Conservation Dependent under the EPBC Act. A biodiversity summary for this region is also available. For more information please see: www.environment.gov.au/heritage/anhat/index.html Limitations • ANHAT currently contains information on the distribution of over 30,000 Australian taxa. This includes all mammals, birds, reptiles, frogs and fish, 137 families of vascular plants (over 15,000 species) and a range of invertebrate groups. Groups notnot yet yet covered covered in inANHAT ANHAT are notnot included included in in the the list. list. • The data used come from authoritative sources, but they are not perfect. All species names have been confirmed as valid species names, but it is not possible to confirm all species locations.