Real Entire Functions with Real Zeros and a Conjecture of Wiman
Total Page:16
File Type:pdf, Size:1020Kb
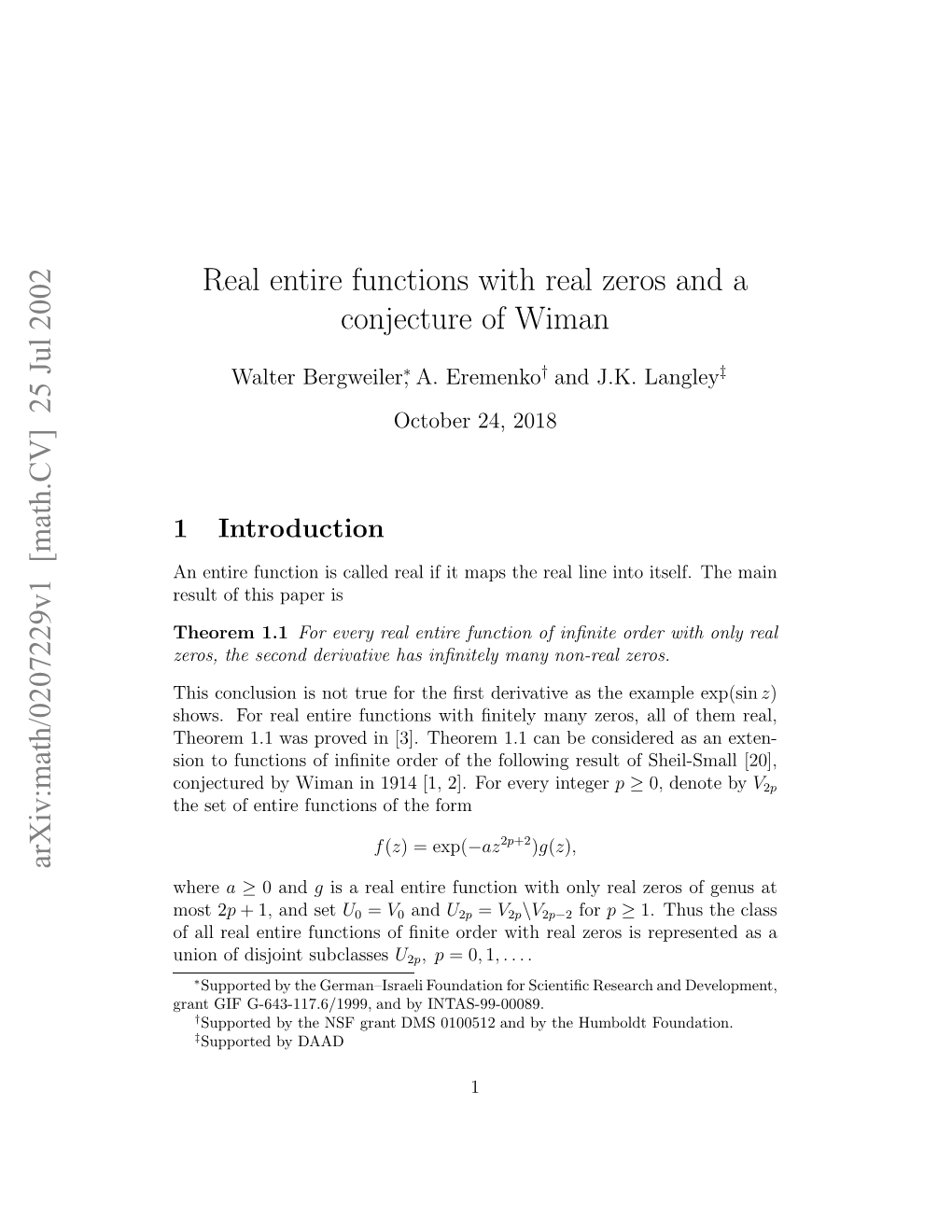
Load more
Recommended publications
-
Which Moments of a Logarithmic Derivative Imply Quasiinvariance?
Doc Math J DMV Which Moments of a Logarithmic Derivative Imply Quasi invariance Michael Scheutzow Heinrich v Weizsacker Received June Communicated by Friedrich Gotze Abstract In many sp ecial contexts quasiinvariance of a measure under a oneparameter group of transformations has b een established A remarkable classical general result of AV Skorokhod states that a measure on a Hilb ert space is quasiinvariant in a given direction if it has a logarithmic aj j derivative in this direction such that e is integrable for some a In this note we use the techniques of to extend this result to general oneparameter families of measures and moreover we give a complete char acterization of all functions for which the integrability of j j implies quasiinvariance of If is convex then a necessary and sucient condition is that log xx is not integrable at Mathematics Sub ject Classication A C G Overview The pap er is divided into two parts The rst part do es not mention quasiinvariance at all It treats only onedimensional functions and implicitly onedimensional measures The reason is as follows A measure on R has a logarithmic derivative if and only if has an absolutely continuous Leb esgue density f and is given by 0 f x ae Then the integrability of jj is equivalent to the Leb esgue x f 0 f jf The quasiinvariance of is equivalent to the statement integrability of j f that f x Leb esgueae Therefore in the case of onedimensional measures a function allows a quasiinvariance criterion as indicated in the abstract -
A Logarithmic Derivative Lemma in Several Complex Variables and Its Applications
TRANSACTIONS OF THE AMERICAN MATHEMATICAL SOCIETY Volume 363, Number 12, December 2011, Pages 6257–6267 S 0002-9947(2011)05226-8 Article electronically published on June 27, 2011 A LOGARITHMIC DERIVATIVE LEMMA IN SEVERAL COMPLEX VARIABLES AND ITS APPLICATIONS BAO QIN LI Abstract. We give a logarithmic derivative lemma in several complex vari- ables and its applications to meromorphic solutions of partial differential equa- tions. 1. Introduction The logarithmic derivative lemma of Nevanlinna is an important tool in the value distribution theory of meromorphic functions and its applications. It has two main forms (see [13, 1.3.3 and 4.2.1], [9, p. 115], [4, p. 36], etc.): Estimate (1) and its consequence, Estimate (2) below. Theorem A. Let f be a non-zero meromorphic function in |z| <R≤ +∞ in the complex plane with f(0) =0 , ∞. Then for 0 <r<ρ<R, 1 2π f (k)(reiθ) log+ | |dθ 2π f(reiθ) (1) 0 1 1 1 ≤ c{log+ T (ρ, f) + log+ log+ +log+ ρ +log+ +log+ +1}, |f(0)| ρ − r r where k is a positive integer and c is a positive constant depending only on k. Estimate (1) with k = 1 was originally due to Nevanlinna, which easily implies the following version of the lemma with exceptional intervals of r for meromorphic functions in the plane: 1 2π f (reiθ) (2) + | | { } log iθ dθ = O log(rT(r, f )) , 2π 0 f(re ) for all r outside a countable union of intervals of finite Lebesgue measure, by using the Borel lemma in a standard way (see e.g. -
Understanding Diagnostic Plots for Well-Test Interpretation
Published in Hydrogeology Journal 17, issue 3, 589-600, 2009 1 which should be used for any reference to this work Understanding diagnostic plots for well-test interpretation Philippe Renard & Damian Glenz & Miguel Mejias Keywords Hydraulic testing . Conceptual models . Hydraulic properties . Analytical solutions Abstract In well-test analysis, a diagnostic plot is a models in order to infer the hydraulic properties of the aquifer. scatter plot of both drawdown and its logarithmic In that framework, a diagnostic plot (Bourdet et al. derivative versus time. It is usually plotted in log–log 1983) is a simultaneous plot of the drawdown and the scale. The main advantages and limitations of the method logarithmic derivative ðÞ@s=@ ln t ¼ t@s=@t of the draw- are reviewed with the help of three hydrogeological field down as a function of time in log–log scale. This plot is examples. Guidelines are provided for the selection of an used to facilitate the identification of an appropriate appropriate conceptual model from a qualitative analysis conceptual model best suited to interpret the data. of the log-derivative. It is shown how the noise on the The idea of using the logarithmic derivative in well-test drawdown measurements is amplified by the calculation interpretation is attributed to Chow (1952). He demon- of the derivative and it is proposed to sample the signal in strated that the transmissivity of an ideal confined aquifer order to minimize this effect. When the discharge rates are is proportional to the ratio of the pumping rate by the varying, or when recovery data have to be interpreted, the logarithmic derivative of the drawdown at late time. -
Symmetric Logarithmic Derivative of Fermionic Gaussian States
entropy Article Symmetric Logarithmic Derivative of Fermionic Gaussian States Angelo Carollo 1,2,* ID , Bernardo Spagnolo 1,2,3 ID and Davide Valenti 1,4 ID 1 Department of Physics and Chemistry, Group of Interdisciplinary Theoretical Physics, Palermo University and CNISM, Viale delle Scienze, Ed. 18, I-90128 Palermo, Italy; [email protected] (B.S.); [email protected] (D.V.) 2 Department of Radiophysics, Lobachevsky State University of Nizhni Novgorod, 23 Gagarin Avenue, Nizhni Novgorod 603950, Russia 3 Istituto Nazionale di Fisica Nucleare, Sezione di Catania, Via S. Sofia 64, I-90123 Catania, Italy 4 Istituto di Biomedicina ed Immunologia Molecolare (IBIM) “Alberto Monroy”, CNR, Via Ugo La Malfa 153, I-90146 Palermo, Italy * Correspondence: [email protected] Received: 21 May 2018; Accepted: 16 June 2018; Published: 22 June 2018 Abstract: In this article, we derive a closed form expression for the symmetric logarithmic derivative of Fermionic Gaussian states. This provides a direct way of computing the quantum Fisher Information for Fermionic Gaussian states. Applications range from quantum Metrology with thermal states to non-equilibrium steady states with Fermionic many-body systems. Keywords: quantum metrology; Fermionic Gaussian state; quantum geometric information 1. Introduction Quantum metrology or quantum parameter estimation is the theory that studies the accuracy by which a physical parameter of a quantum system can be estimated through measurements and statistical inference. In many physical scenarios, quantities which are to be estimated may not be directly observable, either due to experimental limitations or on account of fundamental principles. When this is the case, one needs to infer the value of the variable after measurements on a given probe. -
Differential Algebraic Equations from Definability
Differential algebraic equations from definability Thomas Scanlon 24 October 2014 Thomas Scanlon ADEs from definability Is the logarithm a function? × The exponential function exp : C ! C has a many-valued analytic × inverse log : C ! C where log is well-defined only up to the adding an element of 2πiZ. Thomas Scanlon ADEs from definability The logarithmic derivative Treating exp and log as functions on functions does not help: If U × is some connected Riemann surface and f : U ! C is analytic, then we deduce a “function” log(f ): U ! C. However, because log(f ) is well-defined up to an additive constant, d @ log(f ) := dz (log(f )) is a well defined function. That is, for M = M (U) the differential field of meromorphic functions on U we have a well-defined differential-analytic function @ log : M× ! M. f 0 Of course, one computes that @ log(f ) = f is, in fact, differential algebraic. Thomas Scanlon ADEs from definability Explanation? Why is the logarithmic differential algebraic? What a silly question! The logarithm is the very first transcendental function whose derivative is computed in a standard calculus course. The differential algebraicity of d dz (log(f )) is merely a consequence of an elementary calculation. The usual logarithmic derivative is an instance of Kolchin’s general theory of algebraic logarithmic derivatives on algebraic groups in which the differential algebraicity is explained by the triviality of the tangent bundle of an algebraic group. It can also be seen as an instance of the main theorem to be discussed today: certain kinds of differential analytic functions constructed from covering maps are automatically differential algebraic due to two key ideas from logic: elimination of imaginaries and the Peterzil-Starchenko theory of o-minimal complex analysis. -
Dictionary of Mathematical Terms
DICTIONARY OF MATHEMATICAL TERMS DR. ASHOT DJRBASHIAN in cooperation with PROF. PETER STATHIS 2 i INTRODUCTION changes in how students work with the books and treat them. More and more students opt for electronic books and "carry" them in their laptops, tablets, and even cellphones. This is This dictionary is specifically designed for a trend that seemingly cannot be reversed. two-year college students. It contains all the This is why we have decided to make this an important mathematical terms the students electronic and not a print book and post it would encounter in any mathematics classes on Mathematics Division site for anybody to they take. From the most basic mathemat- download. ical terms of Arithmetic to Algebra, Calcu- lus, Linear Algebra and Differential Equa- Here is how we envision the use of this dictio- tions. In addition we also included most of nary. As a student studies at home or in the the terms from Statistics, Business Calculus, class he or she may encounter a term which Finite Mathematics and some other less com- exact meaning is forgotten. Then instead of monly taught classes. In a few occasions we trying to find explanation in another book went beyond the standard material to satisfy (which may or may not be saved) or going curiosity of some students. Examples are the to internet sources they just open this dictio- article "Cantor set" and articles on solutions nary for necessary clarification and explana- of cubic and quartic equations. tion. The organization of the material is strictly Why is this a better option in most of the alphabetical, not by the topic. -
Reminder. Vector and Matrix Differentiation, Integral Formulas
Euler equations for multiple integrals January 22, 2013 Contents 1 Reminder of multivariable calculus 2 1.1 Vector differentiation . 2 1.2 Matrix differentiation . 3 1.3 Multidimensional integration . 7 1 This part deals with multivariable variational problems that describe equi- libria and dynamics of continua, optimization of shapes, etc. We consideration of a multivariable domain x 2 Ω ⊂ Rd and coordinates x = (x1; : : : ; xd). The variational problem requires minimization of an integral over Ω of a Lagrangian L(x; u; Du) where u = u(x) is a multivariable vector function and ru of mini- mizers and matrix Du = ru of gradients of these minimizers. The optimality conditions for these problems include differentiation with respect to vectors and matrices. First, we recall several formulas of vector (multivariable) calculus which will be commonly used in the following chapters. 1 Reminder of multivariable calculus 1.1 Vector differentiation We remind the definition of vector derivative or derivative of a scalar function with respect to a vector argument a 2 Rn. Definition 1.1 If φ(a) is a scalar function of a column vector argument a = T dφ (a1; : : : ; an) , then the derivative da is a row vector 0 a 1 dφ dφ dφ 1 = ;:::; if a = @ ::: A (1) da da1 dan an assuming that all partial derivatives exist. This definition comes from consideration of the differential dφ of φ(a): dφ(a) dφ(a) = φ(a + da) − φ(a) = · da + o(kak) da Indeed, the left-hand side is a scalar and the second multiplier in the right-hand side is a column vector, therefore the first multiplier is a row vector defined in (1) Examples of vector differentiation The next examples show the calcula- tion of derivative for several often used functions. -
Official Worksheet 5: the Logarithmic Derivative and the Argument Principle
Official Worksheet 5: The Logarithmic derivative and the argument principle. April 8, 2020 1 Definitions and the argument principle Today's worksheet is a short one: we cover the logarithmic derivative and the argument principle Suppose that f is a meromorphic function on a domain Ω (recall: this is a function which is either holomorphic on Ω or is singular with only isolated singularities, all of which are poles.) Define the logarithmic derivative d log f 0 f := : dz f Note that for any nonzero function f; this definition makes sense everywhere except zeroes or poles of f (where it has at worst poles), since f 0 is defined everywhere that f is. The notation d log is indicative of the fact that, where d d log defined, dx log(f(x)) = dz f: The logarithmic derivative d log has a number of nice properties. They key property is sometimes known as the argument principle, and is as follows. f 0 Theorem 1. 1. If f is nonzero and nonsingular at z0; then f is nonsingular at z0: f 0 2. If f has a pole of order n at z0 then f has a simple pole with residue equal to −n at z0: f 0 3. If f has a zero of degree n at z0 then f has a simple pole with residue equal to n at z0: Corollary 1. If f is a meromorphic function on Ω and γ is a simple closed H f 0 curve inside Ω such that f is nonsingular and nowhere zero on γ; then γ f (z)dz is equal to 2πi(Z − P ); where Z is the number of zeroes of f inside γ and P is the number of poles inside of γ, both counted with multiplicity (i.e. -
A Note on the Logarithmic Derivative of the Gamma Function
THE EDINBURGH MATHEMATICAL NOTES PUBLISHED BY THE EDINBURGH MATHEMATICAL SOCIETY EDITED BY D. MARTIN, M.A., B.Sc, Ph.D. No. 38 1952 A note on the logarithmic derivative of the gamma function By A. P. GUINAND. Introduction. The object of this note is to give simpler proof* of two formulae involving the function <p (z) which I have proved elsewhere by more complicated methods.1 The formulae are 2 ^. (x + 1) - log x = 2J" U (t + 1) - log t) cos 2nxt dt (1> for all real positive x, and for | arg z | < TT, where y is Euler's constant. Proof of (I). Now3 1 Journal London Math. Soc., 22 (1947), 14-18. f{z) denotes lv (.-;) / Y (z). 'J The first formula shows that ^{x + 1) - log x is self-reciprocal with respect to the Fourier cosine kernel 2 cos 2TTX. It is strange that this result should have been overlooked, but I can find no trace of it. Cf. B. M. Mehrotra, Journal Indian Math. Soc. (New Series), 1 (1934), 209-27 for a list of self-reciprocal functions and references. 8 C. A. Stewart, Advanced Calculus, (London, 1940), 495 and 497. Downloaded from https://www.cambridge.org/core. IP address: 170.106.40.139, on 26 Sep 2021 at 06:18:02, subject to the Cambridge Core terms of use, available at https://www.cambridge.org/core/terms. https://doi.org/10.1017/S0950184300002949 A. P. GUINAND __ _. d^ Also dt Jo by Frullani's integral,1 and o t2 + x* 2x Combining these results with (3) and (4) we have JO tdt (5) \2T7< etirt — 1 Hence 2 f'C|i/;(<+ 1)—logiUos Jol J u e — f»/l 1 \ fM = 2 - — u , Idtt c~ "'cos 2nxtdt )0\u e ~ll Jo J 0 \u e« - _ 2 -1 * J o \ 2irt • eZnt — ' = ip (x + 1) — log £ by (5). -
Timath.Com Calculus
TImath.com Calculus The Logarithmic Derivative Time required ID: 9092 45 minutes Activity Overview Students will determine the derivative of the function y = ln(x) and work with the derivative of both y = ln(u) and y = loga(u). In the process, the students will show that ln(a+− h) ln(a) 1 lim = . h0→ ha Topic: Formal Differentiation • Derive the Logarithmic Rule and the Generalized Logarithmic Rule for differentiating logarithmic functions. • Prove that ln( x )= ln(a) ⋅ loga ( x ) by graphing f(x) = loga(x) and g(x) = ln(x) for some a and deduce the Generalized Rule for Logarithmic differentiation. • Apply the rules for differentiating exponential and logarithmic functions. Teacher Preparation and Notes • This investigation derives the definition of the logarithmic derivative. The students should be familiar with keystrokes for the limit command, the derivative command, entering the both the natural logarithmic and the general logarithmic functions, drawing a graph, and setting up and displaying a table. • Before starting this activity, students should go to the home screen and select F6:Clean Up > 2:NewProb, and then press . This will clear any stored variables, turn off any functions and plots, and clear the drawing and home screens. • This activity is designed to be student-centered with the teacher acting as a facilitator while students work cooperatively. • To download the student worksheet, go to education.ti.com/exchange and enter “9092” in the keyword search box. Associated Materials • LogarithmicDerivative_Student.doc Suggested Related Activities To download any activity listed, go to education.ti.com/exchange and enter the number in the keyword search box. -
3 Additional Derivative Topics and Graphs
3 Additional Derivative Topics and Graphs 3.1 The Constant e and Continuous Compound Interest The Constant e The number e is defined as: 1 n lim 1 + = lim(1 + s)1=s = 2:718281828459 ::: x!1 n s!0 Continuous Compound Interest If a principal P is invested at an annual rate r (expressed as a decimal) compounded continuously, then the amount A in the account at the end of t years is given by the compound interest formula: A = P ert 3.2 Derivatives of Exponential and Logarithmic Functions Derivatives of Exponential Functions For b > 0, b 6= 1: d • ex = ex dx d • bx = bx ln b dx Derivatives of Logarithmic Functions For b > 0, b 6= 1, and x > 0: d 1 • ln x = dx x d 1 1 • log x = dx b ln b x 1 Change-of-Base Formulas The change-of-base formulas allow conversion from base e to any base b, b > 0, b 6= 1: • bx = ex ln b ln x • log x = b ln b 3.3 Derivatives of Products and Quotients Product Rule If y = f(x) = F (x)S(x), then f 0(x) = F (x)S0(x) + S(x)F 0(x) provided that both F 0(x) and S0(x) exist. Quotient Rule T (x) If y = f(x) = , then B(x) B(x)T 0(x) − T (x)B0(x) f 0(x) = [B(x)]2 provided that both T 0(x) and B0(x) exist. 3.4 The Chain Rule Composite Functions A function m is a composite of functions f and g if m = f[g(x)]. -
Introduction to Differential Equations
Derivatives – An Introduction Concepts of primary interest: Definition: first derivative Differential of a function Left-side and right-side limits Higher derivatives Mean value and Rolle’s theorem L’Hospital Rule for indeterminate forms Implicit differentiation Inverse functions Logarithmic Derivative Method for Products Extrema and the Determinant Test for functions of two variables Lagrange Undetermined Multipliers (*** needs serious edit ****) Sample calculations: 2 Derivative of x Chain Rule Product Rule Saddle-points and local extrema Derivative of the inverse function for Squared d(sinθ) /dθ = 1 for θ = 0 Applications Closest point of approach or CPA (nautical term) Tools of the Trade Derivatives of inverse functions Contact: [email protected] 11/1/2012 Plotting inverse functions ***ADD a table of the derivatives of common functions See Tipler; Lagrange Approximating Functions Many functions are complicated, and their evaluations require detailed, tortuous calculations. To avoid the stress, approximate representations are sometimes substituted for the actual functions of interest. For a continuous function f(x), it might be adequate to replace the function in a small interval around x0 by its value at that point f(x0). A better approximation for a function f(x) with a continuous derivative follows from the definition of that derivative. df fx()− fx ( ) = Limit 0 [DI.1] x0 xx→ dx 0 x− x0 df df Clearly, fx()≅+ fx ( ) ( x − x) where is the slope of the function at x0.. 00dx dx x0 x0 11/1/2012 Physics Handout Series.Tank: Derivatives D/DX- 2 Figure DI-1 This handout formalizes the definition of a derivative and illustrates a few basic properties and applications of the derivative.