The Phase Diagram of Water and the Magnetic Fields of Uranus And
Total Page:16
File Type:pdf, Size:1020Kb
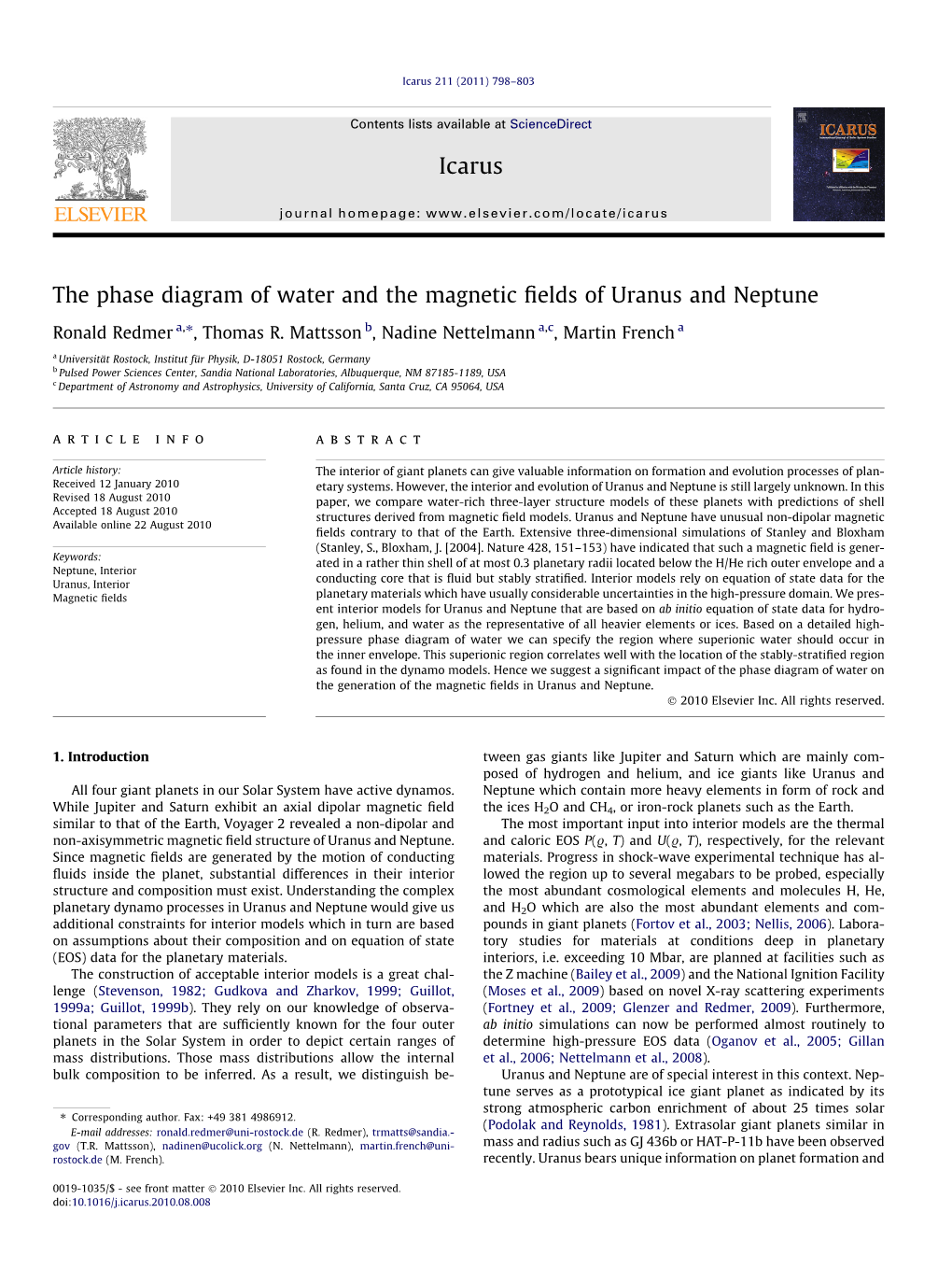
Load more
Recommended publications
-
Business of the City Council City of Mercer Island, Wa
AB 5444 BUSINESS OF THE CITY COUNCIL July 17, 2018 CITY OF MERCER ISLAND, WA Study Session REVIEW RFQ CRITERIA FOR TRANSIT Action: Discussion Only COMMUTER PARKING AND A PUBLIC- Review proposed RFQ criteria and Action Needed: PRIVATE, MIXED-USE DEVELOPMENT selection process. Motion Ordinance PROJECT ON THE TULLY’S/PARCEL 12 Resolution SITE DEPARTMENT OF City Manager (Julie Underwood) COUNCIL LIAISON n/a EXHIBITS 1. Request For Qualifications (RFQ) - Mercer Island Commuter Parking & Town Center Mixed-Use Project 2018-2019 CITY COUNCIL GOAL 1. Prepare for Light Rail/Improve Mobility APPROVED BY CITY MANAGER AMOUNT OF EXPENDITURE $ n/a AMOUNT BUDGETED $ n/a APPROPRIATION REQUIRED $ n/a SUMMARY At its meeting on June 5, the City Council authorized the City Manager to execute a Purchase and Sale Agreement with the Parkway Management Group, et al. to acquire the former Tully’s property, located at 7810 SE 27th Street (see AB 5434). This property will be combined with a portion of adjacent land the City already owns at Sunset Highway, known as Parcel 12. These properties could be developed through a public-private partnership to build an underground, transit commuter parking facility and potential mixed-use development (see AB 5418). The April 2018 Citizen Survey (see AB 5440) showed that 59% of respondents were unsatisfied with the availability of commuter parking, and the majority of respondents selected commuter parking as their top transportation priority. This public-private partnership presents an opportunity to provide much-needed commuter parking in Town Center, while significantly reducing the City’s contribution of funds (other than the Sound Transit contribution) by utilizing City-owned land in a key geographic location near the future East Link light rail station. -
First Experimental Evidence for Superionic Ice 6 February 2018
First experimental evidence for superionic ice 6 February 2018 In a paper published today by Nature Physics, a research team from Lawrence Livermore National Laboratory (LLNL), the University of California, Berkeley and the University of Rochester provides experimental evidence for superionic conduction in water ice at planetary interior conditions, verifying the 30-year-old prediction. Using shock compression, the team identified thermodynamic signatures showing that ice melts near 5000 Kelvin (K) at 200 gigapascals (GPa—2 million times Earth's atmosphere)—4000 K higher than the melting point at 0.5 megabar (Mbar) and almost the surface temperature of the sun. Time-integrated image of a laser-driven shock compression experiment to recreate planetary interior conditions and study the properties of superionic water. "Our experiments have verified the two main Credit: M. Millot/E. Kowaluk/J.Wickboldt/LLNL/LLE/NIF predictions for superionic ice: very high protonic/ionic conductivity within the solid and high melting point," said lead author Marius Millot, a physicist at LLNL. "Our work provides experimental Among the many discoveries on matter at high evidence for superionic ice and shows that these pressure that garnered him the Nobel Prize in predictions were not due to artifacts in the 1946, scientist Percy Bridgman discovered five simulations, but actually captured the extraordinary different crystalline forms of water ice, ushering in behavior of water at those conditions. This provides more than 100 years of research into how ice an important validation of state-of-the-art quantum behaves under extreme conditions. simulations using density-functional-theory-based molecular dynamics (DFT-MD)." One of the most intriguing properties of water is that it may become superionic when heated to "Driven by the increase in computing resources several thousand degrees at high pressure, similar available, I feel we have reached a turning point," to the conditions inside giant planets like Uranus added Sebastien Hamel, LLNL physicist and co- and Neptune. -
Download File
International Journal of Current Advanced Research ISSN: O: 2319-6475, ISSN: P: 2319-6505, Impact Factor: 6.614 Available Online at www.journalijcar.org Volume 7; Issue 6(I); June 2018; Page No. 13658-13661 DOI: http://dx.doi.org/10.24327/ijcar.2018.13661.2450 Research Article FLUORIDE INCIDENCE IN GROUNDWATER AND ITS POTENTIAL HEALTH EFFECTS IN PARTS OF BILASPURAREA, CHHATTISGARH, INDIA Manish Upadhyay and Raman C.V University Kargi Road, Kota Bilaspur (C.G) ARTICLE INFO ABSTRACT Article History: Although fluoride was once considered an essential nutrient, the U.S. National Research Council has since removed this designation due to the lack of studies showing it is essential Received 20th March, 2018 th for human growth, though still considering fluoride a "beneficial element" due to its Received in revised form 27 positive impact on oral health. The U.S. specifies the optimal level of fluoride to range April, 2018 Accepted 5th May, 2018 th from 0.7 to 1.2 mg/L (milligrams per liter, equivalent to parts per million), depending on Published online 28 June, 2018 the average maximum daily air temperature; the optimal level is lower in warmer climates, where people drink more water, and is higher in cooler climates.High concentrations of Key words: fluoride (F-) in drinking water are harmful to human health.This communication reports F- incidence in groundwaterand its relation with the prevalence of dental and skeletal Climate, dosage, fluorosis,permissible fluorosisin Sarguja , Chhattisgarh,India. In 1994 a World Health Organization expert limit,consumption. committee on fluoride use stated that 1.0 mg/L should be an absolute upper bound, even in cold climates, and that 0.5 mg/L may be an appropriate lower limit A 2007 Australian systematic review recommended a range from 0.6 to 1.1 mg/L Assayof fluoride concentration in ground water samples around in some parts of Bilaspur revealed that fluoride content in beyond the permissible limit in a some residential areas. -
Bulloch Times (Statesboro News-Statesboro Eagle)
Georgia Southern University Digital Commons@Georgia Southern Bulloch County Newspapers (Single Issues) Bulloch County Historical Newspapers 1-5-1928 Bulloch Times (Statesboro News-Statesboro Eagle) Notes Condition varies. Some pages missing or in poor condition. Originals provided for filming by the publisher. Gift of tS atesboro Herald and the Bulloch County Historical Society. Follow this and additional works at: https://digitalcommons.georgiasouthern.edu/bulloch-news- issues Recommended Citation "Bulloch Times (Statesboro News-Statesboro Eagle)" (1928). Bulloch County Newspapers (Single Issues). 1360. https://digitalcommons.georgiasouthern.edu/bulloch-news-issues/1360 This newspaper is brought to you for free and open access by the Bulloch County Historical Newspapers at Digital Commons@Georgia Southern. It has been accepted for inclusion in Bulloch County Newspapers (Single Issues) by an authorized administrator of Digital Commons@Georgia Southern. For more information, please contact [email protected]. (STATESBORO NEW �- STATESBOR ) EAGLE) STATESBORO. GA., THURSDAY, JM�. 5, 1928 CHfVROUT COM NY :��i;;;�i�f¥�:;:;::;�;;j STAHS80RO MAR�H STAusmo' Bll'iiYtfIWlHVnAR'S FREEZI nOELS wheels as standard equ.ip!nent. Other 8RINGS ABUNDANT ICE PRESENTS NEW standard equipment includes stop- GETS (NDORSfMENT MARINES CARAGU�' " When the weather forecnstel' Sun- rear vision BETTER" CAR light, mirror, gasoline AUTHORITY SPEAKS "BIGGER AND TOBACCO the of the se RECORDS DUCLOSE gauge, complete tool set, and theft day foretold. coming EXA«. HAS MANY NEW ADVAN. WORD OF ENCOURAGEMENT COST " vorcst cold of the season, with tem OF PROOUCTIOIII' FOIt. proo steering and lock. Ad TAGES AND REFINEMENTS., ignition TO STATESBORO. perature to below 20. he MEMBERS OF 6-ACRE CLUB•• ditional oquipment is standard with likely drop hulf the truth-c-for individual models. -
Review of Research Impact Factor : 5.2331(Uif) Ugc Approved Journal No
Review Of ReseaRch impact factOR : 5.2331(Uif) UGc appROved JOURnal nO. 48514 issn: 2249-894X vOlUme - 7 | issUe - 9 | JUne - 2018 __________________________________________________________________________________________________________________________ HIGH FLUORIDE INCIDENCE IN GROUNDWATER AND ITS POTENTIAL HEALTH EFFECTS IN PARTS OF SARGUJA AREA, CHHATTISGARH Dr. Manish Upadhyay HOD & Associate Professor, Dr. C.V. Raman University, Kota, Bilaspur, Chattisgarh. ABSTRACT : Assay of fluoride concentration in ground water samples around Surajpur district in Sarguja revealed that fluoride content in beyond the permissible limit in some residential areas. The extent of Fluoride present in different samples was obtained by spectrophotometer. the extent of fluoride was found in village Baraul found to be from minimum 2.4 to 3.0 mg/l. village Ramtirath found to be from minimum 2.1 to 3.0 mg/l. but in village Fatehpur and Barwahi found to be from minimum 2.1 to 3.50 mg/l. it is further added that extent of fluoride content in water depends on the climatic conditions and increase in summer. KEYWORDS : Fluoridation, Dosage, Fluorosis, Aesthetic, Consumption. INTRODUCTION Safe drinking water is essential to humans and other life forms. Access to safe drinking water has improved over the last decades in almost every part of the world, but approximately one billion people still lack access to safe water and over 2.5 billion lack accesses to adequate sanitation. There is a clear correlation between access to safe water and GDP per capita. However, some observers have estimated that by 2025 more than half of the world population will be facing water-based vulnerability. A recent report (November 2009) suggests that by 2030, in some developing regions of the world, water demand will exceed supply by 50%.Water plays an important role in the world economy, as it functions as a solvent for a wide variety of chemical substances and facilitates industrial cooling and transportation. -
Plastic and Superionic Helium Ammonia Compounds Under High Pressure and High Temperature
PHYSICAL REVIEW X 10, 021007 (2020) Featured in Physics Plastic and Superionic Helium Ammonia Compounds under High Pressure and High Temperature Cong Liu,1 Hao Gao,1 Andreas Hermann,2 Yong Wang,1 Maosheng Miao,3 Chris J. Pickard,4,5 Richard J. Needs,6 Hui-Tian Wang,1 Dingyu Xing,1 and Jian Sun 1,* 1National Laboratory of Solid State Microstructures, School of Physics and Collaborative Innovation Center of Advanced Microstructures, Nanjing University, Nanjing, 210093, China 2Centre for Science at Extreme Conditions and The School of Physics and Astronomy, The University of Edinburgh, Peter Guthrie Tait Road, Edinburgh EH9 3FD, United Kingdom 3Department of Chemistry and Biochemistry, California State University Northridge, Northridge, California 91330-8262, USA 4Department of Materials Science & Metallurgy, University of Cambridge, 27 Charles Babbage Road, Cambridge CB3 0FS, United Kingdom 5Advanced Institute for Materials Research, Tohoku University 2-1-1 Katahira, Aoba, Sendai, 980-8577, Japan 6Theory of Condensed Matter Group, Cavendish Laboratory, J.J. Thomson Avenue, Cambridge CB3 0HE, United Kingdom (Received 25 November 2019; revised manuscript received 25 February 2020; accepted 10 March 2020; published 9 April 2020) Both helium and ammonia are main components of icy giant planets. While ammonia is very reactive, helium is the most inert element in the universe. It is of great interest whether ammonia and helium can react with each other under planetary conditions, and if so, what kinds of structures and states of matter can form. Here, using crystal structure prediction methods and first-principles calculations, we report three new stable stoichiometries and eight new stable phases of He-NH3 compounds under pressures up to 500 GPa. -
Helium Compounds at High Pressure ✉ ✉ Jingming Shi 1, Wenwen Cui 1, Jian Hao1,2, Meiling Xu1, Xianlong Wang 3 & Yinwei Li 1
ARTICLE https://doi.org/10.1038/s41467-020-16835-z OPEN Formation of ammonia–helium compounds at high pressure ✉ ✉ Jingming Shi 1, Wenwen Cui 1, Jian Hao1,2, Meiling Xu1, Xianlong Wang 3 & Yinwei Li 1 Uranus and Neptune are generally assumed to have helium only in their gaseous atmo- spheres. Here, we report the possibility of helium being fixed in the upper mantles of these planets in the form of NH3–He compounds. Structure predictions reveal two energetically – 1234567890():,; stable NH3 He compounds with stoichiometries (NH3)2He and NH3He at high pressures. At − low temperatures, (NH3)2He is ionic with NH3 molecules partially dissociating into (NH2) + and (NH4) ions. Simulations show that (NH3)2He transforms into intermediate phase at 100 GPa and 1000 K with H atoms slightly vibrate around N atoms, and then to a superionic phase at ~2000 K with H and He exhibiting liquid behavior within the fixed N sublattice. Finally, (NH3)2He becomes a fluid phase at temperatures of 3000 K. The stability of (NH3)2He at high pressure and temperature could contribute to update models of the interiors of Uranus and Neptune. 1 Laboratory of Quantum Materials Design and Application, School of Physics and Electronic Engineering, Jiangsu Normal University, Xuzhou 221116, China. 2 Jiangsu Key Laboratory of Advanced Laser Materials and Devices, Jiangsu Normal University, Xuzhou 221116, China. 3 Key Laboratory of Materials Physics, ✉ Institute of Solid State Physics, Chinese Academy of Sciences, Hefei 230031, China. email: [email protected]; [email protected] NATURE COMMUNICATIONS | (2020) 11:3164 | https://doi.org/10.1038/s41467-020-16835-z | www.nature.com/naturecommunications 1 ARTICLE NATURE COMMUNICATIONS | https://doi.org/10.1038/s41467-020-16835-z nowledge of the interior compositions of planets is crucial moments of the ice giants. -
Ab Initio Simulations of Superionic H2O, H2O2, and H9O4 Compounds
Ab initio Simulations of Superionic H2O, H2O2, and H9O4 Compounds Burkhard Militzer1,2,a) and Shuai Zhang1,3 1Department of Earth and Planetary Science, University of California, Berkeley, CA 94720, USA 2Department of Astronomy, University of California, Berkeley, CA 94720, USA 3Lawrence Livermore National Laboratory, Livermore, California 94550, USA a)Corresponding author: [email protected] Abstract. Using density functional molecular dynamics simulations, we study the behavior of different hydrogen-oxygen com- pounds at megabar pressures and several thousands of degrees Kelvin where water has been predicted to occur in superionic form. When we study the close packed hcp and dhcp structures of superionic water, we find that they have comparable Gibbs free en- ergies to the fcc structure that we predicted previously [Phys. Rev. Lett., 110 (2013) 151102]. Then we present a comprehensive comparison of different superionic water candidate structures with P21, P21/c, P3121, Pcca, C2/m, and Pa3¯ symmetry that are based on published ground-state structures. We find that the P21 and P21/c structures transform into a different superionic structure with P2 /c symmetry, which at 4000 K has a lower Gibbs free energy than fcc for pressures higher than 22.8 0.5 Mbar. This 1 ± novel structure may also be obtained by distorting a hcp supercell. Finally we show that H2O2 and H9O4 structures will also assume a superionic state at elevated temperatures. Based on Gibbs free energy calculations at 5000 K, we predict that superionic water decompose into H O and H O at 68.7 0.5 Mbar. 2 2 9 4 ± INTRODUCTION Water is one of the most prevalent substances in the solar system [1]. -
Open Questions on the Structures of Crystalline Water Ices ✉ Thomas Loerting 1 , Violeta Fuentes-Landete1,2, Christina M
COMMENT https://doi.org/10.1038/s42004-020-00349-2 OPEN Open questions on the structures of crystalline water ices ✉ Thomas Loerting 1 , Violeta Fuentes-Landete1,2, Christina M. Tonauer 1 & Tobias M. Gasser1 1234567890():,; Water can form a vast number of topological frameworks owing to its hydrogen- bonding ability, with 19 different forms of ice experimentally confirmed at pre- sent. Here, the authors comment on open questions and possible future dis- coveries, covering negative to ultrahigh pressures. The hydrogen bond as envisioned by Linus Pauling is key to the structures of H2O ices. A hydrogen bond can be characterised as O–H⋯O, where the hydrogen atom is linked via a short covalent bond to the donor oxygen and via the longer bond to the acceptor. The one feature that makes ice structures so diverse is that four hydrogen bonds can be formed by a water molecule, although it has only three atoms. Thereby, two atoms may act as H donors, two as H acceptors. In ice, as opposed to water clusters or liquid water, all four hydrogen bonds are developed, resulting in a tetrahedral coordination around each water molecule. This local coordination motif is called the Walrafen pentamer and is found in almost all ice structures1. Hydrogen bonding at ultrahigh pressure Figure 1 summarises the phase diagram for water in a very broad pressure regime, up to pressures as high as encountered in the interior of the ice giants, Uranus and Neptune. Only at ultrahigh pressures, exceeding 50 GPa, the Walrafen pentamer breaks down. In this pressure regime, the hydrogen atom becomes centred between the two oxygen atoms. -
Euopean Journal of Molecular Biology and Biochemistry
Manish Upadhyay. / European Journal of Molecular Biology and Biochemistry. 2014;1(1):7-12. e - ISSN - 2348 - 2206 Print ISSN - 2348 - 2192 European Journal of Molecular Biology and Biochemistry Journal homepage: www.mcmed.us/journal/ejmbb ANALYTICAL STUDY OF FLUORIDE ION CONCENTRATION IN THE DRINKING WATER OF BODLA BLOCK IN KABEERDHAM DISTRICT, CHHATTISGARH Manish Upadhyay Department of Chemistry, C.V.Raman University, Kota, Bilaspur, Chattisgarh, India. ARTICLE INFO A B S T R A C T Received 25/08/2013 Although fluoride was once considered an essential nutrient, the U.S. National Research Revised 15/09/2013 Council has since removed this designation due to the lack of studies showing it is essential Accepted 18/10/2013 for human growth, though still considering fluoride a "beneficial element" due to its positive impact on oral health. The U.S. specifies the optimal level of fluoride to range Key words: from 0.7 to 1.2 mg/L (milligrams per liter, equivalent to parts per million), depending on Fluoridation, Dosage, the average maximum daily air temperature; the optimal level is lower in warmer climates, Fluorosis, Aesthetic, where people drink more water, and is higher in cooler. In 1994 a World Health Consumption. Organization expert committee on fluoride use stated that 1.0 mg/L should be an absolute upper bound, even in cold climates, and that 0.5 mg/L may be an appropriate lower limit A 2007 Australian systematic review recommended a range from 0.6 to 1.1 mg/L. Fluoride's adverse effects depend on total fluoride dosage from all sources. -
Depleting Groundwater Levels and Increasing Fluoride Concentration in Villages of Surajpur District, Chhattisgarh, India: Cost to Economy and Health
IOSR Journal of Applied Chemistry (IOSRJAC) ISSN : 2278-5736 Volume 1, Issue 5 (July-Aug 2012), PP 15-22 www.iosrjournals.org Depleting Groundwater Levels And Increasing Fluoride Concentration In Villages Of Surajpur District, Chhattisgarh, India: Cost To Economy And Health. Dr. Manish Upadhyay HOD & Associate Professor, Dr.C.V.Raman University Kargi Road ,KotaBilaspur (C.G) Abstract: Although fluoride was once considered an essential nutrient, the U.S. National Research Council has since removed this designation due to the lack of studies showing it is essential for human growth, though still considering fluoride a "beneficial element" due to its positive impact on oral health. The U.S. specifies the optimal level of fluoride to range from 0.7 to 1.2 mg/L (milligrams per liter, equivalent to parts per million), depending on the average maximum daily air temperature; the optimal level is lower in warmer climates, where people drink more water, and is higher in cooler climates The U.S. standard, adopted in 1962, is not appropriate for all parts of the world and is based on assumptions that have become obsolete with the rise of air conditioning and increased use of soft drinks, processed food, and other sources of fluorides. In 1994 a World Health Organization expert committee on fluoride use stated that 1.0 mg/L should be an absolute upper bound, even in cold climates, and that 0.5 mg/L may be an appropriate lower limit A 2007 Australian systematic review recommended a range from 0.6 to 1.1 mg/L. A 2000 systematic review found that water fluoridation was statistically associated with a decreased proportion of children with cavities (the median of mean decreases was 14.6%, the range −5 to 64%), and with a decrease in decayed, missing, and filled primary teeth (the median of mean decreases was 2.25 teeth, the range 0.5–4.4 teeth), which is roughly equivalent to preventing 40% of cavities. -
Multiple Superionic States in Helium-Water Compounds
Multiple superionic states in helium-water compounds Cong Liu,1 Hao Gao,1 Yong Wang,1 Richard J. Needs,2 Chris J. Pickard,3, 4 Jian Sun,1, ∗ Hui-Tian Wang,1, y and Dingyu Xing1 1National Laboratory of Solid State Microstructures, School of Physics and Collaborative Innovation Center of Advanced Microstructures, Nanjing University, Nanjing, 210093, P. R. China 2Theory of Condensed Matter Group, Cavendish Laboratory, J J Thomson Avenue, Cambridge CB3 0HE, UK 3Department of Materials Science & Metallurgy, University of Cambridge, 27 Charles Babbage Road, Cambridge CB3 0FS, UK 4Advanced Institute for Materials Research, Tohoku University 2-1-1 Katahira, Aoba, Sendai, 980-8577, Japan (Dated: May 23, 2019) Superionic states are phases of matter that can simultaneously exhibit some of the properties of a liquid and of a solid. For example, in superionic ice, hydrogen atoms can move freely while oxygen atoms are fixed in their sublattice. \Superionicity" has attracted much attention both in fundamental science and applications. Helium is the most inert element in nature and it is generally considered to be unreactive. Here we use ab initio calculations to show that He and H2O can form stable compounds within a large pressure range which can exist even close to ambient pressure. Surprisingly, we find that they can form two previously unknown types of superionic states. In the first of these phases the helium atoms exhibit liquid behavior within a fixed ice-lattice framework. In the second of these phases, both helium and hydrogen atoms move in a liquid-like fashion within a fixed oxygen sublattice. Because the He-O interaction is weaker than the H-O interaction, the helium atoms in these superionic states have larger diffusion coefficients and lower \melting" temperatures than that of hydrogen, although helium is heavier than hydrogen.