Ecological Studies of Benthic Macroinvertebrates for Determining Sedimentation Impacts In
Total Page:16
File Type:pdf, Size:1020Kb
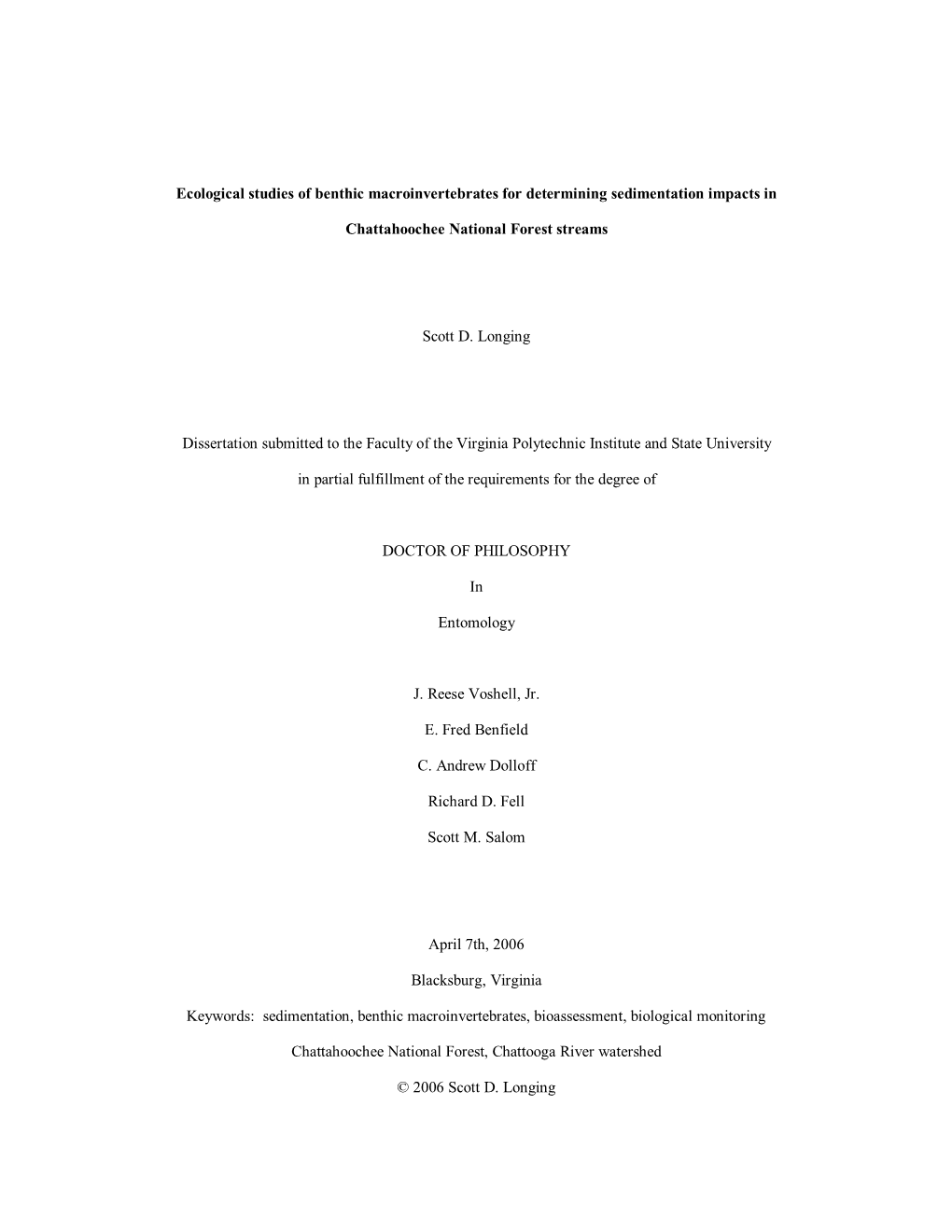
Load more
Recommended publications
-
ARTHROPOD COMMUNITIES and PASSERINE DIET: EFFECTS of SHRUB EXPANSION in WESTERN ALASKA by Molly Tankersley Mcdermott, B.A./B.S
Arthropod communities and passerine diet: effects of shrub expansion in Western Alaska Item Type Thesis Authors McDermott, Molly Tankersley Download date 26/09/2021 06:13:39 Link to Item http://hdl.handle.net/11122/7893 ARTHROPOD COMMUNITIES AND PASSERINE DIET: EFFECTS OF SHRUB EXPANSION IN WESTERN ALASKA By Molly Tankersley McDermott, B.A./B.S. A Thesis Submitted in Partial Fulfillment of the Requirements for the Degree of Master of Science in Biological Sciences University of Alaska Fairbanks August 2017 APPROVED: Pat Doak, Committee Chair Greg Breed, Committee Member Colleen Handel, Committee Member Christa Mulder, Committee Member Kris Hundertmark, Chair Department o f Biology and Wildlife Paul Layer, Dean College o f Natural Science and Mathematics Michael Castellini, Dean of the Graduate School ABSTRACT Across the Arctic, taller woody shrubs, particularly willow (Salix spp.), birch (Betula spp.), and alder (Alnus spp.), have been expanding rapidly onto tundra. Changes in vegetation structure can alter the physical habitat structure, thermal environment, and food available to arthropods, which play an important role in the structure and functioning of Arctic ecosystems. Not only do they provide key ecosystem services such as pollination and nutrient cycling, they are an essential food source for migratory birds. In this study I examined the relationships between the abundance, diversity, and community composition of arthropods and the height and cover of several shrub species across a tundra-shrub gradient in northwestern Alaska. To characterize nestling diet of common passerines that occupy this gradient, I used next-generation sequencing of fecal matter. Willow cover was strongly and consistently associated with abundance and biomass of arthropods and significant shifts in arthropod community composition and diversity. -
New Species and Records of Chimarra (Trichoptera, Philopotamidae) from Northeastern Brazil, and an Updated Key to Subgenus Ch
A peer-reviewed open-access journal ZooKeys 491: 119–142 (2015) New species of Chimarra from Northeastern Brazil 119 doi: 10.3897/zookeys.491.8553 RESEARCH ARTICLE http://zookeys.pensoft.net Launched to accelerate biodiversity research New species and records of Chimarra (Trichoptera, Philopotamidae) from Northeastern Brazil, and an updated key to subgenus Chimarra (Chimarrita) Albane Vilarino1, Adolfo Ricardo Calor1 1 Universidade Federal da Bahia, Instituto de Biologia, Departamento de Zoologia, PPG Diversidade Animal, Laboratório de Entomologia Aquática - LEAq. Rua Barão de Jeremoabo, 147, campus Ondina, Ondina, CEP 40170-115, Salvador, Bahia, Brazil Corresponding authors: Albane Vilarino ([email protected]); Adolfo Ricardo Calor ([email protected]) Academic editor: R. Holzenthal | Received 4 September 2014 | Accepted 12 February 2015 | Published 26 March 2015 http://zoobank.org/E6E62707-9A0F-477D-ACE9-B02553171FBD Citation: Vilarino A, Calor AR (2015) New species and records of Chimarra (Trichoptera, Philopotamidae) from Northeastern Brazil, and an updated key to subgenus Chimarra (Chimarrita). ZooKeys 491: 119–142. doi: 10.3897/ zookeys.491.8553 Abstract Two new species of Chimarra (Chimarrita) are described and illustrated, Chimarra (Chimarrita) mesodonta sp. n. and Chimarra (Chimarrita) anticheira sp. n. from the Chimarra (Chimarrita) rosalesi and Chimarra (Chimarrita) simpliciforma species groups, respectively. The morphological variation ofChimarra (Curgia) morio is also illustrated. Chimarra (Otarrha) odonta and Chimarra (Chimarrita) kontilos are reported to occur in the northeast region of Brazil for the first time. An updated key is provided for males and females of the all species in the subgenus Chimarrita. Keywords Biodiversity, caddisflies, Curgia, description, Neotropics, phylogenetic relationships, taxonomy Introduction Philopotamidae Stephens, 1829 is a cosmopolitan family with approximately 1,270 de- scribed species in 19 extant genera. -
Species Fact Sheet for Homoplectra Schuhi
SPECIES FACT SHEET Common Name: Schuh’s Homoplectran Caddisfly Scientific Name: Homoplectra schuhi Denning 1965 Phylum: Mandibulata Class: Insecta Order: Trichoptera Suborder: Annulipalpia Family: Hydropsychidae Subfamily: Diplectroninae Conservation Status Global Status (2005): G3Q – Vulnerable, but taxonomic questions persist (last reviewed 25 Mar 2005) National Status (United States): N3 - Vulnerable (23 Feb 2005) State Status (Oregon): S3 - Vulnerable (NatureServe 2015) Oregon Biodiversity Information Center: List 3 IUCN Red List: NE – Not evaluated Taxonomic Note This species has been given a global status of G3Q due to the limited number of specimens that have been reviewed to date, and the variability of diagnostic characteristics (NatureServe 2015). This genus is in need of additional collecting and taxonomic review, which may lead to synonymization with older described species (Wisseman 2015, Ruiter 2015). For example, specimens identified as H. luchia Denning 1966 may in fact be synonyms of H. schuhi (Ruiter 2015). Technical Description A microscope is required to identify Homoplectra schuhi, as identifications are based on genitalia anatomy. The advice of a Trichoptera expert is suggested. See Denning (1965) for lateral view drawings of the male and female genitalia. Adult: The adults of this species are small, moth-like insects in the caddisfly family Hydropsychidae. Homoplectra males are recognized by the complexity of the phallic apparatus, which can be complicated by very strong development of several sclerotized branches (Schmid 1998). Holotype male: Length 6 mm. General color of head, thorax and abdomen dark brown, wings tan with no pattern, legs and antennae varying shades of brownish. Pubescence of head, thorax and legs aureous. Fifth sternite with a dorsal filament enlarged distally and curved dorso-caudad. -
Data Quality, Performance, and Uncertainty in Taxonomic Identification for Biological Assessments
J. N. Am. Benthol. Soc., 2008, 27(4):906–919 Ó 2008 by The North American Benthological Society DOI: 10.1899/07-175.1 Published online: 28 October 2008 Data quality, performance, and uncertainty in taxonomic identification for biological assessments 1 2 James B. Stribling AND Kristen L. Pavlik Tetra Tech, Inc., 400 Red Brook Blvd., Suite 200, Owings Mills, Maryland 21117-5159 USA Susan M. Holdsworth3 Office of Wetlands, Oceans, and Watersheds, US Environmental Protection Agency, 1200 Pennsylvania Ave., NW, Mail Code 4503T, Washington, DC 20460 USA Erik W. Leppo4 Tetra Tech, Inc., 400 Red Brook Blvd., Suite 200, Owings Mills, Maryland 21117-5159 USA Abstract. Taxonomic identifications are central to biological assessment; thus, documenting and reporting uncertainty associated with identifications is critical. The presumption that comparable results would be obtained, regardless of which or how many taxonomists were used to identify samples, lies at the core of any assessment. As part of a national survey of streams, 741 benthic macroinvertebrate samples were collected throughout the eastern USA, subsampled in laboratories to ;500 organisms/sample, and sent to taxonomists for identification and enumeration. Primary identifications were done by 25 taxonomists in 8 laboratories. For each laboratory, ;10% of the samples were randomly selected for quality control (QC) reidentification and sent to an independent taxonomist in a separate laboratory (total n ¼ 74), and the 2 sets of results were compared directly. The results of the sample-based comparisons were summarized as % taxonomic disagreement (PTD) and % difference in enumeration (PDE). Across the set of QC samples, mean values of PTD and PDE were ;21 and 2.6%, respectively. -
( ) Hydropsychidae (Insecta: Trichoptera) As Bio-Indicators Of
ว.วิทย. มข. 40(3) 654-666 (2555) KKU Sci. J. 40(3) 654-666 (2012) แมลงน้ําวงศ!ไฮดรอบไซคิดี้ (อันดับไทรคอบเทอร-า) เพื่อเป2นตัวบ-งชี้ทางชีวภาพของคุณภาพน้ํา Hydropsychidae (Insecta: Trichoptera) as Bio-indicators of Water QuaLity แตงออน พรหมมิ1 บทคัดยอ การประเมินคุณภาพน้ําในแมน้ําและลําธารควรที่จะมีการใชปจจัยทางกายภาพ เคมีและชีวภาพควบคูกัน ไป ปจจัยทางชีวภาพที่มีศักยภาพในการประเมินคุณภาพน้ําในแหลงน้ําคือกลุมสัตว+ไมมีกระดูกสันหลังขนาดใหญที่ อาศัยอยูตามพื้นทองน้ํา โดยเฉพาะแมลงน้ําอันดับไทรคอบเทอรา ซึ่งเป3นกลุมสัตว+ที่มีความหลากหลายมากกลุม หนึ่งในแหลงน้ํา ระยะตัวออนของแมลงกลุมนี้ทุกชนิดอาศัยอยูในแหลงน้ํา เป3นองค+ประกอบหลักในแหลงน้ําและ เป3นตัวหมุนเวียนสารอาหารในแหลงน้ํา ระยะตัวออนของแมลงน้ํากลุมนี้จะตอบสนองตอปจจัยของสภาพแวดลอม ในแหลงน้ําทุกรูปแบบ ระยะตัวเต็มวัยอาศัยอยูบนบกบริเวณตนไมซึ่งไมไกลจากแหลงน้ํามากนัก หากินเวลา กลางคืน ความรูทางดานอนุกรมวิธานและชีววิทยาไมวาจะเป3นระยะตัวออนหรือตัวเต็มวัยของแมลงน้ําอันดับไทร คอบเทอราในประเทศแถบยุโรปตะวันตกและอเมริกาเหนือสามารถวินิจฉัยไดถึงระดับชนิด โดยเฉพาะแมลงน้ํา วงศ+ไฮดรอบไซคิดี้ มีการประยุกต+ใชในการติดตามตรวจสอบทางชีวภาพของคุณภาพน้ํา เนื่องจากชนิดของตัวออน แมลงน้ําวงศ+นี้มีความทนทานตอมลพิษในชวงกวางมากกวาแมลงน้ําชนิดอื่น ๆ 1สายวิชาวิทยาศาสตร+ คณะศิลปศาสตร+และวิทยาศาสตร+ มหาวิทยาลัยเกษตรศาสตร+ วิทยาเขตกําแพงแสน จ.นครปฐม 73140 E-mail: [email protected] บทความ วารสารวิทยาศาสตร+ มข. ปQที่ 40 ฉบับที่ 3 655 ABSTRACT Assessment on rivers and streams water quality should incorporate aspects of chemical, physical, and biological. Of all the potential groups of freshwater organisms that have been considered for -
Insecta: Trichoptera) from the Ecoregion Hellenic Western Balkans
Nat. Croat. Vol. 26(2), 2017 197 NAT. CROAT. VOL. 26 No 2 197-204 ZAGREB DECEMBER 31, 2017 original scientific paper / izvorni znanstveni rad DOI 10.20302/NC.2017.26.16 FIRST RECORD OF TRIAENODES BICOLOR (CURTIS, 1834) (INSECTA: TRICHOPTERA) FROM THE ECOREGION HELLENIC WESTERN BALKANS Halil Ibrahimi1, Ruzhdi Kuçi2*, Astrit Bilalli3 & Ermira Gashi1 1Department of Biology, Faculty of Mathematical and Natural Sciences, University of Prishtina “Hasan Prishtina”, “Mother Theresa” p.n., 10 000 Prishtinë, Republic of Kosovo 2Faculty of Education, University of Prishtina “Hasan Prishtina”, “Mother Teresa” p.n., 10 000 Prishtinë, Republic of Kosovo 3Faculty of Agribusiness, University of Peja “Haxhi Zeka”, “UÇK” street, 30 000 Pejë, Republic of Kosovo Ibrahimi, H., Kuçi, R., Bilalli, A. & Gashi, E.: First record of Triaenodes bicolor (Curtis, 1834) (Insecta: Trichoptera) from the Ecoregion Hellenic Western Balkans. Nat. Croat., Vol. 26, No. 2., 197-204, Zagreb, 2017. We collected adult caddisfly specimens with entomological nets and ultraviolet light traps monthly from May to November 2012 in Brezne Lake situated in Dragash Municipality. During this investigation we found the Leptocerid species Triaenodes bicolor for the first time in Kosovo; it is also the first record for Ecoregion 6, Hellenic Western Balkans. Additionally, this is the first record of the genus Triaenodes from Kosovo. In total seven males and three females of this species were found. Triaenodes bicolor is present all over the European continent but has been rarely sampled in southeastern Europe. Other taxa sympatric with Triaenodes bicolor in the investigated locality are: Hydropsyche instabilis, Hydropsyche spp., Plectrocnemia conspersa, Plectrocnemia spp., Micropterna nycterobia, Micropterna sequax, Limnephilus vittatus, Limnephilus auricula and Thremma anomalum. -
Aquatic Versus Terrestrial Insects: Real Or Presumed Differences in Population Dynamics?
insects Review Aquatic versus Terrestrial Insects: Real or Presumed Differences in Population Dynamics? Jill Lancaster * and Barbara J. Downes School of Geography, University of Melbourne, Melbourne, VIC 3010, Australia; [email protected] * Correspondence: [email protected]; Tel.: +61-3-834-40917 Received: 12 October 2018; Accepted: 30 October 2018; Published: 1 November 2018 Abstract: The study of insect populations is dominated by research on terrestrial insects. Are aquatic insect populations different or are they just presumed to be different? We explore the evidence across several topics. (1) Populations of terrestrial herbivorous insects are constrained most often by enemies, whereas aquatic herbivorous insects are constrained more by food supplies, a real difference related to the different plants that dominate in each ecosystem. (2) Population outbreaks are presumed not to occur in aquatic insects. We report three examples of cyclical patterns; there may be more. (3) Aquatic insects, like terrestrial insects, show strong oviposition site selection even though they oviposit on surfaces that are not necessarily food for their larvae. A novel outcome is that density of oviposition habitat can determine larval densities. (4) Aquatic habitats are often largely 1-dimensional shapes and this is presumed to influence dispersal. In rivers, drift by insects is presumed to create downstream dispersal that has to be countered by upstream flight by adults. This idea has persisted for decades but supporting evidence is scarce. Few researchers are currently working on the dynamics of aquatic insect populations; there is scope for many more studies and potentially enlightening contrasts with terrestrial insects. Keywords: dispersal; drift; insect flight; herbivory; outbreaks; oviposition; North Atlantic Oscillation; parasites; population cycles; population regulation 1. -
Trichoptera:Hydropsychidae) Based on DNA and Morphological Evidence Christy Jo Geraci National Museum on Natural History, Smithsonian Institute
Clemson University TigerPrints Publications Biological Sciences 3-2010 Defining the Genus Hydropsyche (Trichoptera:Hydropsychidae) Based on DNA and Morphological Evidence Christy Jo Geraci National Museum on Natural History, Smithsonian Institute Xin Zhou University of Guelph John C. Morse Clemson University, [email protected] Karl M. Kjer Rutgers University - New Brunswick/Piscataway Follow this and additional works at: https://tigerprints.clemson.edu/bio_pubs Part of the Biology Commons Recommended Citation Please use publisher's recommended citation. This Article is brought to you for free and open access by the Biological Sciences at TigerPrints. It has been accepted for inclusion in Publications by an authorized administrator of TigerPrints. For more information, please contact [email protected]. J. N. Am. Benthol. Soc., 2010, 29(3):918–933 ’ 2010 by The North American Benthological Society DOI: 10.1899/09-031.1 Published online: 29 June 2010 Defining the genus Hydropsyche (Trichoptera:Hydropsychidae) based on DNA and morphological evidence Christy Jo Geraci1 Department of Entomology, National Museum of Natural History, Smithsonian Institution, Washington, DC 20013-7012 USA Xin Zhou2 Biodiversity Institute of Ontario, University of Guelph, Guelph, Ontario, N1G 2W1 Canada John C. Morse3 Department of Entomology, Soils, and Plant Sciences, Clemson University, Clemson, South Carolina 29634 USA Karl M. Kjer4 Department of Ecology, Evolution and Natural Resources, School of Environmental and Biological Sciences, Rutgers University, New Brunswick, New Jersey 08901 USA Abstract. In this paper, we review the history of Hydropsychinae genus-level classification and nomenclature and present new molecular evidence from mitochondrial cytochrome c oxidase subunit I (COI) and nuclear large subunit ribosomal ribonucleic acid (28S) markers supporting the monophyly of the genus Hydropsyche. -
Species at Risk on Department of Defense Installations
Species at Risk on Department of Defense Installations Revised Report and Documentation Prepared for: Department of Defense U.S. Fish and Wildlife Service Submitted by: January 2004 Species at Risk on Department of Defense Installations: Revised Report and Documentation CONTENTS 1.0 Executive Summary..........................................................................................iii 2.0 Introduction – Project Description................................................................. 1 3.0 Methods ................................................................................................................ 3 3.1 NatureServe Data................................................................................................ 3 3.2 DOD Installations............................................................................................... 5 3.3 Species at Risk .................................................................................................... 6 4.0 Results................................................................................................................... 8 4.1 Nationwide Assessment of Species at Risk on DOD Installations..................... 8 4.2 Assessment of Species at Risk by Military Service.......................................... 13 4.3 Assessment of Species at Risk on Installations ................................................ 15 5.0 Conclusion and Management Recommendations.................................... 22 6.0 Future Directions............................................................................................. -
Ohio EPA Macroinvertebrate Taxonomic Level December 2019 1 Table 1. Current Taxonomic Keys and the Level of Taxonomy Routinely U
Ohio EPA Macroinvertebrate Taxonomic Level December 2019 Table 1. Current taxonomic keys and the level of taxonomy routinely used by the Ohio EPA in streams and rivers for various macroinvertebrate taxonomic classifications. Genera that are reasonably considered to be monotypic in Ohio are also listed. Taxon Subtaxon Taxonomic Level Taxonomic Key(ies) Species Pennak 1989, Thorp & Rogers 2016 Porifera If no gemmules are present identify to family (Spongillidae). Genus Thorp & Rogers 2016 Cnidaria monotypic genera: Cordylophora caspia and Craspedacusta sowerbii Platyhelminthes Class (Turbellaria) Thorp & Rogers 2016 Nemertea Phylum (Nemertea) Thorp & Rogers 2016 Phylum (Nematomorpha) Thorp & Rogers 2016 Nematomorpha Paragordius varius monotypic genus Thorp & Rogers 2016 Genus Thorp & Rogers 2016 Ectoprocta monotypic genera: Cristatella mucedo, Hyalinella punctata, Lophopodella carteri, Paludicella articulata, Pectinatella magnifica, Pottsiella erecta Entoprocta Urnatella gracilis monotypic genus Thorp & Rogers 2016 Polychaeta Class (Polychaeta) Thorp & Rogers 2016 Annelida Oligochaeta Subclass (Oligochaeta) Thorp & Rogers 2016 Hirudinida Species Klemm 1982, Klemm et al. 2015 Anostraca Species Thorp & Rogers 2016 Species (Lynceus Laevicaudata Thorp & Rogers 2016 brachyurus) Spinicaudata Genus Thorp & Rogers 2016 Williams 1972, Thorp & Rogers Isopoda Genus 2016 Holsinger 1972, Thorp & Rogers Amphipoda Genus 2016 Gammaridae: Gammarus Species Holsinger 1972 Crustacea monotypic genera: Apocorophium lacustre, Echinogammarus ischnus, Synurella dentata Species (Taphromysis Mysida Thorp & Rogers 2016 louisianae) Crocker & Barr 1968; Jezerinac 1993, 1995; Jezerinac & Thoma 1984; Taylor 2000; Thoma et al. Cambaridae Species 2005; Thoma & Stocker 2009; Crandall & De Grave 2017; Glon et al. 2018 Species (Palaemon Pennak 1989, Palaemonidae kadiakensis) Thorp & Rogers 2016 1 Ohio EPA Macroinvertebrate Taxonomic Level December 2019 Taxon Subtaxon Taxonomic Level Taxonomic Key(ies) Informal grouping of the Arachnida Hydrachnidia Smith 2001 water mites Genus Morse et al. -
Contribution to the Knowledge of the Caddisfly Fauna (Insecta: Trichoptera) of Leqinat Lakes and Adjacent Streams in Bjeshkët E Nemuna (Kosovo)
View metadata, citation and similar papers at core.ac.uk brought to you by CORE NAT. CROAT. VOL. 28 No 1 35-44 ZAGREB June 30, 2019 original scientific paper / izvorni znanstveni rad DOI 10.20302/NC.2019.28.3 CONTRIBUTION TO THE KNOWLEDGE OF THE CADDISFLY FAUNA (INSECTA: TRICHOPTERA) OF LEQINAT LAKES AND ADJACENT STREAMS IN BJESHKËT E NEMUNA (KOSOVO) Halil Ibrahimi1, Linda Grapci-Kotori1,*, Astrit Bilalli2, Albulena Qamili1 & Robert Schabetsberger3 1Department of Biology, Faculty of Mathematical and Natural Sciences, University of Prishtina, “Mother Theresa” p.n., 10 000 Prishtinë, Kosovo 2Faculty of Agribusiness, University of Peja “Haxhi Zeka”, UÇK street p.n., 30 000 Pejë, Kosovo 3Department of Cell Biology, Faculty of Natural Sciences, University of Salzburg, Hellbrunnerstraße 34, Raum Nr. E-2.050, Salzburg, Austria Ibrahimi, H., Grapci-Kotori, L., Bilalli, A., Qamili, A. & Schabetsberger, R.: Contribution to the knowledge of the caddisfly fauna (Insecta: Trichoptera) of Leqinat lakes and adjacent streams in Bjeshkët e Nemuna (Kosovo). Nat. Croat. Vol. 28, No. 1., 35-44, Zagreb, 2019. Adult caddisflies were collected with entomological nets and ultraviolet light traps during August and September 2018 in Leqinat Lake, Drelaj Lake and five adjacent streams in Bjeshkët e Nemuna in Kosovo. Within the current study we found three first records for the caddisfly fauna of Kosovo: Limnephilus flavospinosus, Limnephilus flavicornis and Oligotricha striata. The genus Oligotricha is reported for the first time from Kosovo. We also found few rare species which have been reported only from few localities in the Balkan Peninsula such as: Plectrocnemia mojkovacensis, Rhyacophila balcanica and Drusus tenellus. -
Plecoptera: Perlidae), with an Annotated Checklist of the Subfamily in the Realm
Opusc. Zool. Budapest, 2016, 47(2): 173–196 On the identity of some Oriental Acroneuriinae taxa (Plecoptera: Perlidae), with an annotated checklist of the subfamily in the realm D. MURÁNYI1 & W.H. LI2 1Dávid Murányi, Department of Civil and Environmental Engineering, Ehime University, Bunkyo-cho 3, Matsuyama, 790-8577 Japan, and Department of Zoology, Hungarian Natural History Museum, H-1088 Budapest, Baross u. 13, Hungary. E-mail: [email protected], [email protected] 2Weihai Li, Department of Plant Protection, Henan Institute of Science and Technology, Xinxiang, Henan, 453003 China. E-mail: [email protected] Abstract. The monotypic Taiwanese genus Mesoperla Klapálek, 1913 is redescribed on the basis of a male syntype specimen, and its affinities are re-evaluated. The single female type specimen of further two Oriental monotypic genera, Kalidasia Klapálek, 1914 and Nirvania Klapálek, 1914, are confirmed to be lost or destroyed respectively; both genera are considered as nomina dubia. The Sichuan endemic Acroneuria grahami Wu & Claassen, 1934 is redescribed on the basis of male holotype. Distinctive characters of the genus Brahmana Klapálek, 1914 consisting of five, inadequately known Oriental species are discussed. Flavoperla needhami (Klapálek, 1916) and Sinacroneuria sinica (Yang & Yang, 1998) comb. novae are suggested for an Indian species originally described in Gibosia Okamoto, 1912 and a Chinese species originally described in Acroneuria Pictet, 1841. At present, 62 species of Acroneuriinae, classified in 10 valid genera are reported from the Oriental Realm but 29 species are inadequately known. A key is presented to distinguish males of the Asian Acroneuriinae genera. Asian distribution of each genera are detailed and depicted on a map.