Gis-Based Approaches to Slope Stability Analysis and Earthquake -Induced Landslide Hazard Zonation
Total Page:16
File Type:pdf, Size:1020Kb
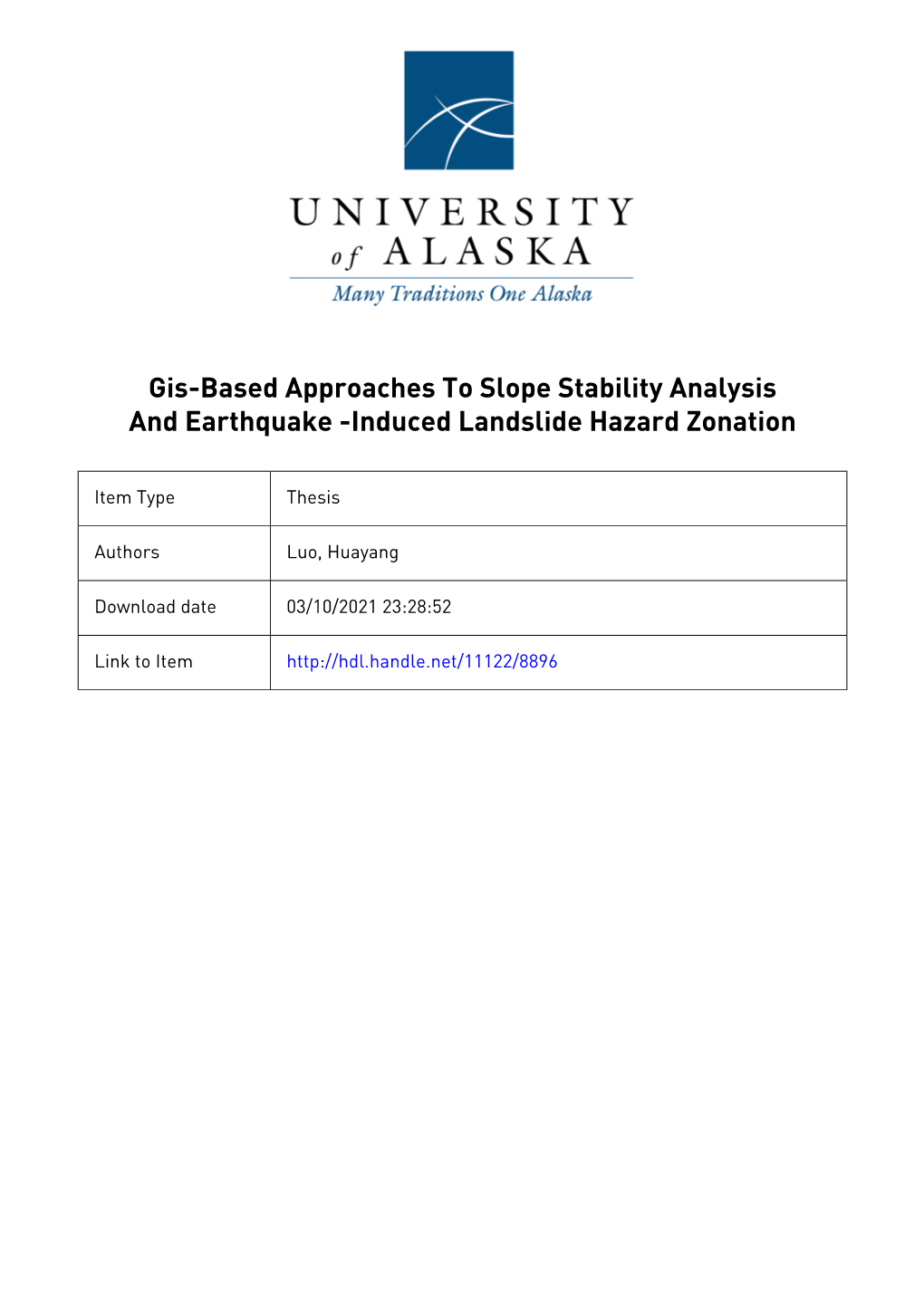
Load more
Recommended publications
-
2021 Oregon Seismic Hazard Database: Purpose and Methods
State of Oregon Oregon Department of Geology and Mineral Industries Brad Avy, State Geologist DIGITAL DATA SERIES 2021 OREGON SEISMIC HAZARD DATABASE: PURPOSE AND METHODS By Ian P. Madin1, Jon J. Francyzk1, John M. Bauer2, and Carlie J.M. Azzopardi1 2021 1Oregon Department of Geology and Mineral Industries, 800 NE Oregon Street, Suite 965, Portland, OR 97232 2Principal, Bauer GIS Solutions, Portland, OR 97229 2021 Oregon Seismic Hazard Database: Purpose and Methods DISCLAIMER This product is for informational purposes and may not have been prepared for or be suitable for legal, engineering, or surveying purposes. Users of this information should review or consult the primary data and information sources to ascertain the usability of the information. This publication cannot substitute for site-specific investigations by qualified practitioners. Site-specific data may give results that differ from the results shown in the publication. WHAT’S IN THIS PUBLICATION? The Oregon Seismic Hazard Database, release 1 (OSHD-1.0), is the first comprehensive collection of seismic hazard data for Oregon. This publication consists of a geodatabase containing coseismic geohazard maps and quantitative ground shaking and ground deformation maps; a report describing the methods used to prepare the geodatabase, and map plates showing 1) the highest level of shaking (peak ground velocity) expected to occur with a 2% chance in the next 50 years, equivalent to the most severe shaking likely to occur once in 2,475 years; 2) median shaking levels expected from a suite of 30 magnitude 9 Cascadia subduction zone earthquake simulations; and 3) the probability of experiencing shaking of Modified Mercalli Intensity VII, which is the nominal threshold for structural damage to buildings. -
Recommended Guidelines for Liquefaction Evaluations Using Ground Motions from Probabilistic Seismic Hazard Analyses
RECOMMENDED GUIDELINES FOR LIQUEFACTION EVALUATIONS USING GROUND MOTIONS FROM PROBABILISTIC SEISMIC HAZARD ANALYSES Report to the Oregon Department of Transportation June, 2005 Prepared by Stephen Dickenson Associate Professor Geotechnical Engineering Group Department of Civil, Construction and Environmental Engineering Oregon State University ODOT Liquefaction Hazard Assessment using Ground Motions from PSHA Page 2 ABSTRACT The assessment of liquefaction hazards for bridge sites requires thorough geotechnical site characterization and credible estimates of the ground motions anticipated for the exposure interval of interest. The ODOT Bridge Design and Drafting Manual specifies that the ground motions used for evaluation of liquefaction hazards must be obtained from probabilistic seismic hazard analyses (PSHA). This ground motion data is routinely obtained from the U.S. Geologocal Survey Seismic Hazard Mapping program, through its interactive web site and associated publications. The use of ground motion parameters derived from a PSHA for evaluations of liquefaction susceptibility and ground failure potential requires that the ground motion values that are indicated for the site are correlated to a specific earthquake magnitude. The individual seismic sources that contribute to the cumulative seismic hazard must therefore be accounted for individually. The process of hazard de-aggregation has been applied in PSHA to highlight the relative contributions of the various regional seismic sources to the ground motion parameter of interest. The use of de-aggregation procedures in PSHA is beneficial for liquefaction investigations because the contribution of each magnitude-distance pair on the overall seismic hazard can be readily determined. The difficulty in applying de-aggregated seismic hazard results for liquefaction studies is that the practitioner is confronted with numerous magnitude-distance pairs, each of which may yield different liquefaction hazard results. -
1. Geology and Seismic Hazards
8 PUBLIC SAFETY ELEMENT As required by State law, the Public Safety Element addresses the protection of the community from unreasonable risks from natural and manmade haz- ards. This Element provides information about risks in the Eden Area and delineates policies designed to prepare and protect the community as much as possible from the effects of: ♦ Geologic hazards, including earthquakes, ground-shaking, liquefaction and landslides. ♦ Flooding, including dam failures and inundation, tsunamis and seiches. ♦ Wildland fires. ♦ Hazardous materials. This Element also contains information and policies regarding general emer- gency preparedness. Each section includes background information on the particular hazard followed by goals, policies and actions designed to minimize risk for Eden Area residents. The Public Safety Element establishes mechanisms to prepare for and reduce death, injuries and damage to property from public safety hazards like flood- ing, fires and seismic events. Hazards are an unavoidable aspect of life, and the Public Safety Element cannot eliminate risk completely. Instead, the Element contains policies to create an acceptable level of risk. 1. GEOLOGY AND SEISMIC HAZARDS Earthquakes and secondary seismic hazards such as ground-shaking, liquefac- tion and landslides are the primary geologic hazards in the Eden Area. This section provides background on the seismic hazards that affect the Eden Area and includes goals, policies and actions to minimize these risks. 8-1 COUNTY OF ALAMEDA EDEN AREA GENERAL PLAN PUBLIC SAFETY ELEMENT A. Background Information As is the case in most of California, the Eden Area is subject to risks from seismic activity. The Eden Area is located in the San Andreas Fault Zone, one of the most seismically-active regions in the United States, which has generated numerous moderate to strong earthquakes in northern California and in the San Francisco Bay Area. -
Bray 2011 Pseudostatic Slope Stability Procedure Paper
Paper No. Theme Lecture 1 PSEUDOSTATIC SLOPE STABILITY PROCEDURE Jonathan D. BRAY 1 and Thaleia TRAVASAROU2 ABSTRACT Pseudostatic slope stability procedures can be employed in a straightforward manner, and thus, their use in engineering practice is appealing. The magnitude of the seismic coefficient that is applied to the potential sliding mass to represent the destabilizing effect of the earthquake shaking is a critical component of the procedure. It is often selected based on precedence, regulatory design guidance, and engineering judgment. However, the selection of the design value of the seismic coefficient employed in pseudostatic slope stability analysis should be based on the seismic hazard and the amount of seismic displacement that constitutes satisfactory performance for the project. The seismic coefficient should have a rational basis that depends on the seismic hazard and the allowable amount of calculated seismically induced permanent displacement. The recommended pseudostatic slope stability procedure requires that the engineer develops the project-specific allowable level of seismic displacement. The site- dependent seismic demand is characterized by the 5% damped elastic design spectral acceleration at the degraded period of the potential sliding mass as well as other key parameters. The level of uncertainty in the estimates of the seismic demand and displacement can be handled through the use of different percentile estimates of these values. Thus, the engineer can properly incorporate the amount of seismic displacement judged to be allowable and the seismic hazard at the site in the selection of the seismic coefficient. Keywords: Dam; Earthquake; Permanent Displacements; Reliability; Seismic Slope Stability INTRODUCTION Pseudostatic slope stability procedures are often used in engineering practice to evaluate the seismic performance of earth structures and natural slopes. -
Seismic Hazards Mapping Act Fact Sheet
Department of Conservation California Geological Survey Seismic Hazards Mapping Act Fact Sheet The Seismic Hazards Mapping Act (SHMA) of 1990 (Public Resources Code, Chapter 7.8, Section 2690-2699.6) directs the Department of Conservation, California Geological Survey to identify and map areas prone to earthquake hazards of liquefaction, earthquake-induced landslides and amplified ground shaking. The purpose of the SHMA is to reduce the threat to public safety and to minimize the loss of life and property by identifying and mitigating these seismic hazards. The SHMA was passed by the legislature following the 1989 Loma Prieta earthquake. Staff geologists in the Seismic Hazard Mapping Program (Program) gather existing geological, geophysical and geotechnical data from numerous sources to compile the Seismic Hazard Zone Maps. They integrate and interpret these data regionally in order to evaluate the severity of the seismic hazards and designate Zones of Required Investigation for areas prone to liquefaction and earthquake–induced landslides. Cities and counties are then required to use the Seismic Hazard Zone Maps in their land use planning and building permit processes. The SHMA requires site-specific geotechnical investigations be conducted identifying the seismic hazard and formulating mitigation measures prior to permitting most developments designed for human occupancy within the Zones of Required Investigation. The Seismic Hazard Zone Maps identify where a site investigation is required and the site investigation determines whether structural design or modification of the project site is necessary to ensure safer development. A copy of each approved geotechnical report including the mitigation measures is required to be submitted to the Program within 30 days of approval of the report. -
Seismic Hazard Analysis and Seismic Slope Stability Evaluation Using Discrete Faults in Northwestern Pakistan
SEISMIC HAZARD ANALYSIS AND SEISMIC SLOPE STABILITY EVALUATION USING DISCRETE FAULTS IN NORTHWESTERN PAKISTAN BY BYUNGMIN KIM DISSERTATION Submitted in partial fulfillment of the requirements for the degree of Doctor of Philosophy in Civil Engineering in the Graduate College of the University of Illinois at Urbana-Champaign, 2012 Urbana, Illinois Doctoral Committee: Professor Youssef M.A. Hashash, Chair, Director of Research Associate Professor Scott M. Olson, Co-director of Research Professor Gholamreza Mesri Professor Amr S. Elnashai Associate Professor Junho Song ABSTRACT The Mw 7.6 earthquake that occurred on 8 October 2005 in Kashmir, Pakistan, resulted in tremendous number of fatalities and injuries, and also triggered numerous landslides. Although there are no reliable means to predict the timing of the earthquake, it is possible to reduce the loss of life and damages associated with strong ground motions and landslides by designing and mitigating structures based on proper seismic hazard and seismic slope stability analyses. This study presents the methodology and results of seismic hazard and slope stability analyses in northwestern Pakistan. The first part of the thesis describes the methodology used to perform deterministic and probabilistic seismic hazard analyses. The methodology of seismic hazard analysis includes identification of seismic sources from 32 faults in NW Pakistan, characterization of recurrence models for the faults based on both historical and instrumented seismicity in addition to geologic evidence, and selection of four plate boundary attenuation relations from the Next Generation Attenuation of Ground Motions Project. Peak ground accelerations for Kaghan and Muzaffarabad which are surrounded by major faults were predicted to be approximately 3 to 4 times greater than estimates by previous studies using diffuse areal source zones. -
Seismic Aspects of Underground Structures of Hydropower Plants
Seismic Aspects of Underground Structures of Hydropower Plants Dr. Martin Wieland Chairman, Committee on Seismic Aspects of Dam Design, International Commission on Large Dams (ICOLD), Poyry Energy Ltd., Hardturmstrasse 161, CH-8037 Zurich, Switzerland [email protected] Abstract Hydropower plants with underground powerhouse contain different types of underground structures namely: diversion tunnels, headrace and tailrace tunnels, access tunnels, caverns grouting and inspection galleries etc. As earthquake ground shaking affects all structures above and below ground at the same time and since some of them must remain operable after the strongest earthquake ground motion, they have to be designed and checked for different types of design earthquakes. The strictest performance criteria apply to spillway and bottom outlet structures, i.e. tunnels, intake, outlet, underground gate structures, etc. In the seismic design of underground structures it must be considered that the earthquake hazard is a mulit-hazard, which includes ground shaking, fault movements, mass movements blocking entrances, intakes and outlets, etc. Underground structures for hydropower plants are mainly in rock, therefore underground structures in soil are not discussed in this paper. Special problems are encountered in the pressure tunnels due to hydrodynamic pressures and leakage of lining of damaged pressure tunnels. The seismic design and performance criteria of underground structures of hydropower plants are discussed in a qualitative way on the basis of the seismic safety criteria applicable to large dams, approved by the International Commission on Large Dams (ICOLD). Keywords: seismic hazard, underground structures, hydropower plants Introduction The general perception of engineers is that underground structures in rock are not vulnerable to earthquakes as tunnels and caverns are assumed to move together with the foundation rock. -
Evaluation of Seismic Performance of Geotechnical Structures
View metadata, citation and similar papers at core.ac.uk brought to you by CORE provided by UC Research Repository Evaluation of seismic performance of geotechnical structures M. Cubrinovski University of Canterbury, Christchurch, New Zealand B. Bradley University of Canterbury, Christchurch, New Zealand ABSTRACT: Three different approaches for assessment of seismic performance of earth structures and soil- structure systems are discussed in this paper. These approaches use different models, analysis procedures and are of vastly different complexity. All three methods are consistent with the performance-based design phi- losophy according to which the seismic performance is assessed using deformational criteria and associated damage. Even though the methods nominally have the same objective, it is shown that they focus on different aspects in the assessment and provide alternative performance measures. Key features of the approaches and their specific contribution in the assessment of geotechnical structures are illustrated using a case study. 1 INTRODUCTION The assessment of seismic performance of geo- Methods for assessment of the seismic performance technical structures is further complicated by uncer- of earth structures and soil-structure systems have tainties and unknowns in the seismic analysis. Par- evolved significantly over the past couple of dec- ticularly significant are the uncertainties associated ades. This involves improvement of both practical with the characterization of deformational behaviour design-oriented approaches and advanced numerical of soils and ground motion itself. Namely, the com- procedures for a rigorous dynamic analysis. In paral- monly encountered lack of geotechnical data for lel with the improved understanding of the physical adequate characterization of the soil profile, in-situ phenomena and overall computational capability, soil conditions and stress-strain behaviour of soils new design concepts have been also developed. -
Area Earthquake Hazards Mapping Project: Seismic and Liquefaction Hazard Maps by Chris H
St. Louis Area Earthquake Hazards Mapping Project: Seismic and Liquefaction Hazard Maps by Chris H. Cramer, Robert A. Bauer, Jae-won Chung, J. David Rogers, Larry Pierce, Vicki Voigt, Brad Mitchell, David Gaunt, Robert A. Wil- liams, David Hoffman, Gregory L. Hempen, Phyllis J. Steckel, Oliver S. Boyd, Connor M. Watkins, Kathleen Tucker, and Natasha S. McCallister ABSTRACT We present probabilistic and deterministic seismic and liquefac- (NMSZ) earthquake sequence. This sequence produced modi- tion hazard maps for the densely populated St. Louis metropolitan fied Mercalli intensity (MMI) for locations in the St. Louis area area that account for the expected effects of surficial geology on that ranged from VI to VIII (Nuttli, 1973; Bakun et al.,2002; earthquake ground shaking. Hazard calculations were based on a Hough and Page, 2011). The region has experienced strong map grid of 0.005°, or about every 500 m, and are thus higher in ground shaking (∼0:1g peak ground acceleration [PGA]) as a resolution than any earlier studies. To estimate ground motions at result of prehistoric and contemporary seismicity associated with the surface of the model (e.g., site amplification), we used a new the major neighboring seismic source areas, including the Wa- detailed near-surface shear-wave velocity model in a 1D equiva- bashValley seismic zone (WVSZ) and NMSZ (Fig. 1), as well as lent-linear response analysis. When compared with the 2014 U.S. a possible paleoseismic earthquake near Shoal Creek, Illinois, Geological Survey (USGS) National Seismic Hazard Model, about 30 km east of St. Louis (McNulty and Obermeier, 1997). which uses a uniform firm-rock-site condition, the new probabi- Another contributing factor to seismic hazard in the St. -
Design of Mechanically Stabilized Earth Wall Connections and End of Walls Subjected to Seismic Loads
Report No. CDOT-2014-1 Final Report DESIGN OF MECHANICALLY STABILIZED EARTH WALL CONNECTIONS AND END OF WALLS SUBJECTED TO SEISMIC LOADDS Panos D. Kiousis Judith Wang Rebecca M. Walthall January 2014 COLORADO DEPARTMENT OF TRANSPORTATION DTD APPLIED RESEARCH AND INNOVATION BRANCH The contents of this report reflect the views of the author(s), who is(are) responsible for the facts and accuracy of the data presented herein. The contents do not necessarily reflect the official views of the Colorado Department of Transportation or the Federal Highway Administration. This report does not constitute a standard, specification, or regulation. Technical Report Documentation Page 1. Report No. 2. Government Accession No. 3. Recipient's Catalog No. CDOT-2014-1 4. Title and Subtitle 5. Report Date DESIGN OF MECHANICALLY STABILIZED EARTH January 2014 WALL CONNECTIONS AND END OF WALLS SUBJECTED 6. Performing Organization Code TO SEISMIC LOADS 7. Author(s) 8. Performing Organization Report No. Panos D. Kiousis, Judith Wang, Rebecca M. Walthall CDOT-2014-1 9. Performing Organization Name and Address 10. Work Unit No. (TRAIS) Colorado School of Mines 1500 Illinois Street 11. Contract or Grant No. Golden, Colorado 80401 74.90 12. Sponsoring Agency Name and Address 13. Type of Report and Period Covered Colorado Department of Transportation - Research Final 4201 E. Arkansas Ave. 14. Sponsoring Agency Code Denver, CO 80222 15. Supplementary Notes Prepared in cooperation with the US Department of Transportation, Federal Highway Administration 16. Abstract The 4th Edition of the AASHTO LRFD Bridge Design Specifications requires all states to design for a 1,000- year return period earthquake, as opposed to earlier editions’ 500-year return period. -
Geotechnical Considerations for Offshore Gravity Type
GEOTECHNICAL CONSIDERATIONS FOR OFFSHORE GRAVITY TYPE STRUCTURES WITH EMPHASIS ON FOUNDATION STABILITY UNDER STORM WAVE LOADING by THOMAS C. GAARD S., The University of California, Davis, 197 A THESIS SUBMITTED IN PARTIAL FULFILMENT OF THE REQUIREMENTS FOR THE DEGREE OF MASTER OF APPLIED SCIENCE in THE FACULTY OF GRADUATE STUDIES DEPARTMENT OF CIVIL ENGINEERING We accept this thesis as conforming to the required standard THE UNIVERSITY OF BRITISH COLUMBIA April, 1982 O Thomas C. Gaard, 1982 In presenting this thesis in partial fulfilment of the requirements for an advanced degree at the University of British Columbia, I agree that the Library shall make it freely available for reference and study. I further agree that permission for extensive copying of this thesis for scholarly purposes may be granted by the head of my department or by his or her representatives. It is understood that copying or publication of this thesis for financial gain shall not be allowed without my written permission. Department of Cl^\ ^^^.,1^ The University of British Columbia 1956 Main Mall Vancouver, Canada V6T 1Y3 Date 32/ ^ J z. % DE-6 (3/81) 11 ABSTRACT A thorough discussion of offshore gravity type structures presently being used, or considered for use in the near future by the oil industry, is presented, along with a brief summary of the major types of structures now used offshore. Factors affecting the stability of offshore gravity type structures are discussed, from the evaluation of a suitable site and the selection of soil parameters, through installation and short-term foundation safety. A case study of the Ekofisk tank is included to show how geotechnical concepts are applied offshore. -
Part I Slope 1 1 Imp.O..R..T.A..N..T
Contents I SLOPE Part I Slope 1 1 Imp.o..r..t.a..n..t. .n..o..t.e..s. ............................................................................................................... 4 2 Dat.a.. .i.m...p..o..r.t. ..................................................................................................................... 5 3 Dat.a.. .e..x..p..o..r.t. ..................................................................................................................... 6 4 Mai.n.. .p..a..r.a..m...e..t.e..r..s. ............................................................................................................. 6 5 Dra.w...i.n..g.. .a..i.d..s. ................................................................................................................... 8 6 Tex.t. .m...a..n..a..g..e..m...e..n..t. ........................................................................................................... 9 7 Pen..e..t.r.a..t.i.o..n.. .t.e..s.t.s.. ............................................................................................................. 9 8 Ins..e..r.t.i.n..g.. .v..e..r.t.i.c..e..s. ........................................................................................................... 10 9 Ou..t.p..u..t. ........................................................................................................................... 11 10 Ge..o..t.e..c.h..n..i.c..a..l. .p..r.o..p..e..r..t.i.e..s. ................................................................................................ 12 Additiona.l.