The Gene Cortex Controls Scale Colour Identity in Heliconius
Total Page:16
File Type:pdf, Size:1020Kb
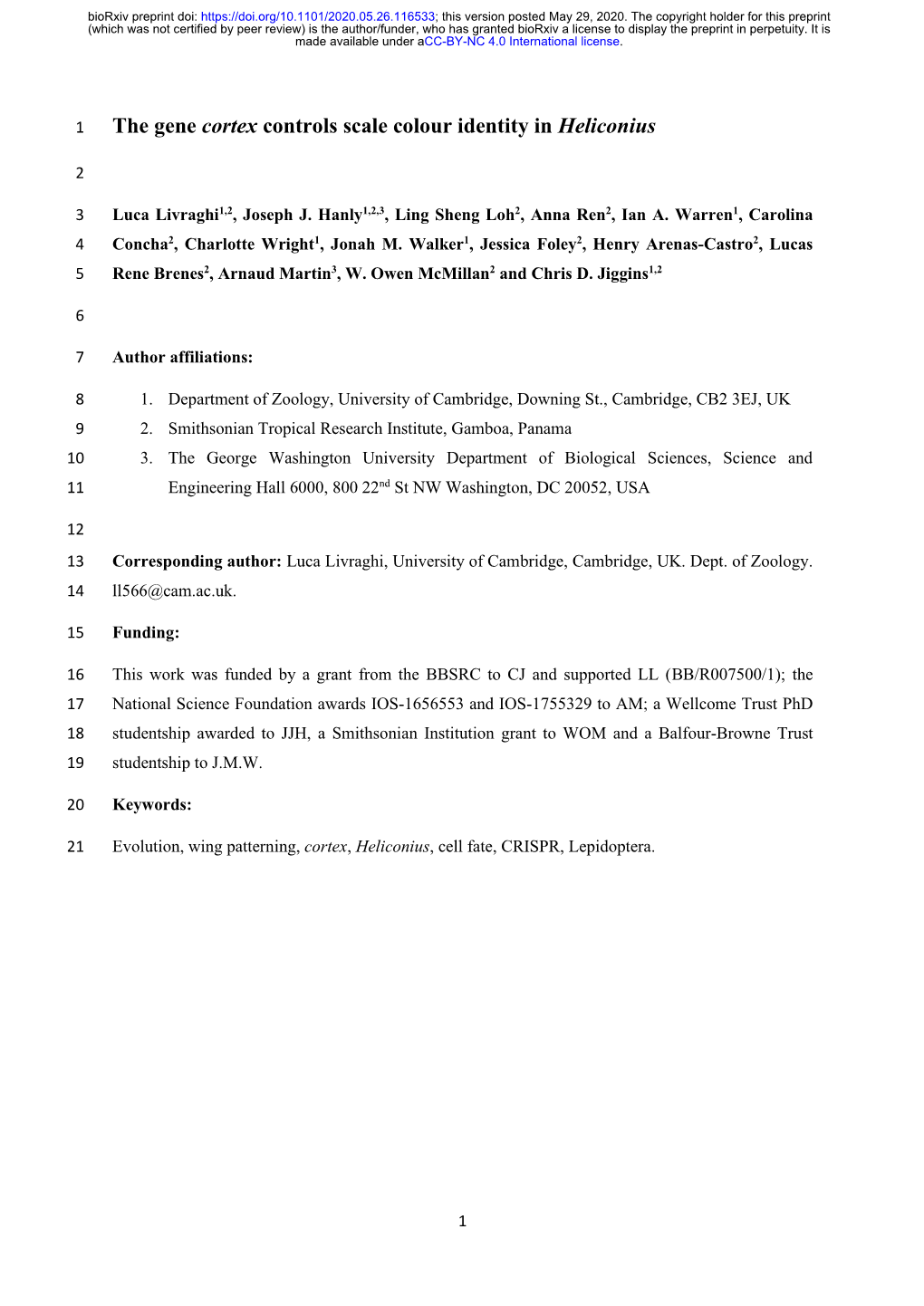
Load more
Recommended publications
-
A Major Locus Controls a Biologically Active Pheromone Component in Heliconius Melpomene
bioRxiv preprint doi: https://doi.org/10.1101/739037; this version posted August 19, 2019. The copyright holder for this preprint (which was not certified by peer review) is the author/funder, who has granted bioRxiv a license to display the preprint in perpetuity. It is made available under aCC-BY-NC 4.0 International license. 1 A major locus controls a biologically active pheromone component in Heliconius melpomene 2 Kelsey J.R.P. Byers1,2,9, Kathy Darragh1,2,9, Jamie Musgrove2, Diana Abondano Almeida2,3, Sylvia Fernanda 3 Garza2,4, Ian A. Warren1, Pasi Rastas5, Marek Kucka6, Yingguang Frank Chan6, Richard M. Merrill7, Stefan 4 Schulz8, W. Owen McMillan2, Chris D. Jiggins1,2,10 5 6 1 Department of Zoology, University of Cambridge, Cambridge, United Kingdom 7 2 Smithsonian Tropical Research Institute, Panama, Panama 8 3 Present address: Institute for Ecology, Evolution and Diversity, Goethe Universität, Frankfurt, Germany 9 4 Present address: Department of Collective Behaviour, Max Planck Institute of Animal Behaviour, 10 Konstanz, Germany & Centre for the Advanced Study of Collective Behaviour, University of Konstanz, 11 Konstanz, Germany 12 5 Institute of Biotechnology, University of Helsinki, Helsinki, Finland 13 6 Friedrich Miescher Laboratory of the Max Planck Society, Tuebingen, Germany 14 7 Division of Evolutionary Biology, Ludwig-Maximilians-Universität München, Munich, Germany 15 8 Institute of Organic Chemistry, Department of Life Sciences, Technische Universität Braunschweig, 16 Braunschweig, Germany 17 9 These authors contributed equally to this work 18 10 To whom correspondence should be addressed: [email protected] 19 Running title: Genetics of bioactive pheromones in Heliconius 20 1 bioRxiv preprint doi: https://doi.org/10.1101/739037; this version posted August 19, 2019. -
The Genetics and Evolution of Iridescent Structural Colour in Heliconius Butterflies
The genetics and evolution of iridescent structural colour in Heliconius butterflies Melanie N. Brien A thesis submitted in partial fulfilment of the requirements for the degree of Doctor of Philosophy The University of Sheffield Faculty of Science Department of Animal & Plant Sciences Submission Date August 2019 1 2 Abstract The study of colouration has been essential in developing key concepts in evolutionary biology. The Heliconius butterflies are well-studied for their diverse aposematic and mimetic colour patterns, and these pigment colour patterns are largely controlled by a small number of homologous genes. Some Heliconius species also produce bright, highly reflective structural colours, but unlike pigment colour, little is known about the genetic basis of structural colouration in any species. In this thesis, I aim to explore the genetic basis of iridescent structural colour in two mimetic species, and investigate its adaptive function. Using experimental crosses between iridescent and non-iridescent subspecies of Heliconius erato and Heliconius melpomene, I show that iridescent colour is a quantitative trait by measuring colour variation in offspring. I then use a Quantitative Trait Locus (QTL) mapping approach to identify loci controlling the trait in the co-mimics, finding that the genetic basis is not the same in the two species. In H. erato, the colour is strongly sex-linked, while in H. melpomene, we find a large effect locus on chromosome 3, plus a number of putative small effect loci in each species. Therefore, iridescence in Heliconius is not an example of repeated gene reuse. I then show that both iridescent colour and pigment colour are sexually dimorphic in H. -
February 2013
If this page does not display correctly, contact Karl Legler at [email protected] Badger ButterFlyer The e-Newsletter of the Southern Wisconsin Butterfly Association JANUARY/FEBRUARY, 2013 WEB SITE: http://www.naba.org/chapters/nabawba/ 1 NEXT SWBA MEETING: Tuesday, April 16 (mark your calendar!) Meet at 7:00 p.m. in Madison at the Warner Park Community Recreation Center, 1625 Northport Drive. Public Programs: 1) Landscaping for Butterflies and other Wildlife (Larry and Emily Scheunemann) In this 30 minute program we will learn about the landscaping of 18 acres of Conservation Reserve Program land (CRP), restored prairie and the creation of a Monarch Waystation. (Tagged Monarchs were recovered in Mexico!) Relax and enjoy pictures of flowers and butterflies as we look forward to another season of both! 2) Dragonflies of Wisconsin: (Karl Legler) A 30 minute program. The 116 Dragonflies of Wisconsin are immensely appealing with their gigantic eyes, intricately veined wings, beautifully colorful bodies, the high-speed flight of an acrobatic aerialist, an insatiable appetite for other flying insects, and a truly fascinating sex life! We will highlight some of the most interesting species in the state. 3) Photo Show and Tell Eastern Amberwing Share some of your favorite butterfly, caterpillar, moth, or dragonfly photos and enjoy the photos of others. You are welcome IN THIS ISSUE: to briefly comment about the subject of your photos. Please 1. NEXT SWBA MEETING email up to 8 digital photos (as file attachments) to Mike Reese at ON TUESDAY, APRIL 16. 2. FIELD TRIPS IN 2013. [email protected] Otherwise, you can bring 3. -
The Speciation History of Heliconius: Inferences from Multilocus DNA Sequence Data
The speciation history of Heliconius: inferences from multilocus DNA sequence data by Margarita Sofia Beltrán A thesis submitted for the degree of Doctor of Philosophy of the University of London September 2004 Department of Biology University College London 1 Abstract Heliconius butterflies, which contain many intermediate stages between local varieties, geographic races, and sympatric species, provide an excellent biological model to study evolution at the species boundary. Heliconius butterflies are warningly coloured and mimetic, and it has been shown that these traits can act as a form of reproductive isolation. I present a species-level phylogeny for this group based on 3834bp of mtDNA (COI, COII, 16S) and nuclear loci (Ef1α, dpp, ap, wg). Using these data I test the geographic mode of speciation in Heliconius and whether mimicry could drive speciation. I found little evidence for allopatric speciation. There are frequent shifts in colour pattern within and between sister species which have a positive and significant correlation with species diversity; this suggests that speciation is facilitated by the evolution of novel mimetic patterns. My data is also consistent with the idea that two major innovations in Heliconius, adult pollen feeding and pupal-mating, each evolved only once. By comparing gene genealogies from mtDNA and introns from nuclear Tpi and Mpi genes, I investigate recent speciation in two sister species pairs, H. erato/H. himera and H. melpomene/H. cydno. There is highly significant discordance between genealogies of the three loci, which suggests recent speciation with ongoing gene flow. Finally, I explore the phylogenetic relationships between races of H. melpomene using an AFLP band tightly linked to the Yb colour pattern locus (which determines the yellow bar in the hindwing). -
Genomic Architecture of Adaptive Color Pattern Divergence and Convergence in Heliconius Butterflies
Downloaded from genome.cshlp.org on September 29, 2021 - Published by Cold Spring Harbor Laboratory Press Research Genomic architecture of adaptive color pattern divergence and convergence in Heliconius butterflies Megan A. Supple,1,2 Heather M. Hines,3,4 Kanchon K. Dasmahapatra,5,6 James J. Lewis,7 Dahlia M. Nielsen,3 Christine Lavoie,8 David A. Ray,8 Camilo Salazar,1,9 W. Owen McMillan,1,10 and Brian A. Counterman8,10,11 1Smithsonian Tropical Research Institute, Panama City, Republic of Panama; 2Biomathematics Program, North Carolina State University, Raleigh, North Carolina 27695, USA; 3Department of Genetics, North Carolina State University, Raleigh, North Carolina 27695, USA; 4Department of Biology, Pennsylvania State University, University Park, Pennsylvania 16802, USA; 5Department of Genetics, Evolution and Environment, University College London, London WC1E 6BT, United Kingdom; 6Department of Biology, University of York, York YO10 5DD, United Kingdom; 7Department of Ecology and Evolutionary Biology, Cornell University, Ithaca, New York 14853, USA; 8Department of Biological Sciences, Mississippi State University, Mississippi State, Mississippi 39762, USA; 9Facultad de Ciencias Naturales y Matema´ticas, Universidad del Rosario, Bogota´ DC, Colombia Identifying the genetic changes driving adaptive variation in natural populations is key to understanding the origins of biodiversity. The mosaic of mimetic wing patterns in Heliconius butterflies makes an excellent system for exploring adaptive variation using next-generation sequencing. In this study, we use a combination of techniques to annotate the genomic interval modulating red color pattern variation, identify a narrow region responsible for adaptive divergence and con- vergence in Heliconius wing color patterns, and explore the evolutionary history of these adaptive alleles. -
Disruptive Sexual Selection Against Hybrids Contributes to Speciation Between Heliconius Cydno and Heliconius Melpomene Russell E
doi 10.1098/rspb.2001.1753 Disruptive sexual selection against hybrids contributes to speciation between Heliconius cydno and Heliconius melpomene Russell E. Naisbit1*, Chris D. Jiggins1,2 and James Mallet1,2 1The Galton Laboratory, Department of Biology, University College London, 4 Stephenson Way, London NW1 2HE, UK 2SmithsonianTropical Research Institute, Apartado 2072, Balboa, Panama Understanding the fate of hybrids in wild populations is fundamental to understanding speciation. Here we provide evidence for disruptive sexual selection against hybrids between Heliconius cydno and Heliconius melpomene. The two species are sympatric across most of Central and Andean South America, and coexist despite a low level of hybridization. No-choice mating experiments show strong assortative mating between the species. Hybrids mate readily with one another, but both sexes show a reduction in mating success of over 50% with the parental species. Mating preference is associated with a shift in the adult colour pattern, which is involved in predator defence through MÏllerian mimicry, but also strongly a¡ects male courtship probability. The hybrids, which lie outside the curve of protection a¡orded by mimetic resemblance to the parental species, are also largely outside the curves of parental mating prefer- ence. Disruptive sexual selection against F1 hybrids therefore forms an additional post-mating barrier to gene £ow, blurring the distinction between pre-mating and post-mating isolation, and helping to main- tain the distinctness of these hybridizing species. Keywords: Lepidoptera; Nymphalidae; hybridization; mate choice; post-mating isolation; pre-mating isolation Rather less experimental work has investigated mate 1. INTRODUCTION choice during speciation and the possibility of the third Studies of recently diverged species are increasingly type of selection against hybrids: disruptive sexual select- producing examples of sympatric species that hybridize in ion. -
Genetic Differentiation Without Mimicry Shift in a Pair of Hybridizing Heliconius Species (Lepidoptera: Nymphalidae)
bs_bs_banner Biological Journal of the Linnean Society, 2013, 109, 830–847. With 5 figures Genetic differentiation without mimicry shift in a pair of hybridizing Heliconius species (Lepidoptera: Nymphalidae) CLAIRE MÉROT1, JESÚS MAVÁREZ2,3, ALLOWEN EVIN4,5, KANCHON K. DASMAHAPATRA6,7, JAMES MALLET7,8, GERARDO LAMAS9 and MATHIEU JORON1* 1UMR CNRS 7205, Muséum National d’Histoire Naturelle, 45 rue Buffon, 75005 Paris, France 2LECA, BP 53, Université Joseph Fourier, 2233 Rue de la Piscine, 38041 Grenoble Cedex, France 3Smithsonian Tropical Research Institute, Apartado 2072, Balboa, Panama 4Department of Archaeology, University of Aberdeen, St. Mary’s Building, Elphinstone Road, Aberdeen AB24 3UF, UK 5UMR CNRS 7209, Muséum National d’Histoire Naturelle, 55 rue Buffon, 75005 Paris, France 6Department of Biology, University of York, Wentworth Way, Heslington, York YO10 5DD, UK 7Department of Genetics, Evolution and Environment, University College London, Gower Street, London WC1E 6BT, UK 8Department of Organismic and Evolutionary Biology, Harvard University, 16 Divinity Avenue, Cambridge, MA 02138, USA 9Museo de Historia Natural, Universidad Nacional Mayor San Marcos, Av. Arenales, 1256, Apartado 14-0434, Lima-14, Peru Received 22 January 2013; revised 25 February 2013; accepted for publication 25 February 2013 Butterflies in the genus Heliconius have undergone rapid adaptive radiation for warning patterns and mimicry, and are excellent models to study the mechanisms underlying diversification. In Heliconius, mimicry rings typically involve distantly related species, whereas closely related species often join different mimicry rings. Genetic and behavioural studies have shown how reproductive isolation in many pairs of Heliconius taxa is largely mediated by natural and sexual selection on wing colour patterns. -
Report of the Tomato Genetics Cooperative
Report of the Tomato Genetics Cooperative Number 54 – September 2004 University of Florida Gulf Coast Research and Education Center 5007 60th Street East Bradenton, FL 34203 USA Foreword The Tomato Genetics Cooperative, initiated in 1951, is a group of researchers who share an interest in tomato genetics, and who have organized informally for the purpose of exchanging information, germplasm, and genetic stocks. The Report of the Tomato Genetics Cooperative is published annually and contains reports of work in progress by members, announcements and updates on linkage maps and materials available. The research reports include work on diverse topics such as new traits or mutants isolated, new cultivars or germplasm developed, interspecific transfer of traits, studies of gene function or control or tissue culture. Relevant work on other Solanaceous species is encouraged as well. Paid memberships currently stand at approximately 145 from 25 countries. Requests for membership (per year) US$15 to addresses in the US and US $20 if shipped to addresses outside of the United States--should be sent to Dr. J.W. Scott, [email protected] (see address information in Announcements section.) Please send only checks or money orders. Make checks payable to the University of Florida. We are sorry but we are NOT able to accept cash, wire transfers or credit cards. Cover photo of Heinz 1706. Heinz 1706 is the tomato variety being sequenced in the worldwide tomato genome project. For further information see report by Rich Ozminkowski on p. 26 who provided the photo. Photo editing by John Petti. - J.W. Scott THIS PAGE IS BLANK IN THE ORIGINAL DOCUMENT Table of Contents Foreward.......................................................................................................................................... -
Variable Selection and the Coexistence of Multiple Mimetic Forms of the Butterfly Heliconius Numata
Evolutionary Ecology 13: 721±754, 1999. Ó 2001 Kluwer Academic Publishers. Printed in the Netherlands. Research paper Variable selection and the coexistence of multiple mimetic forms of the butter¯y Heliconius numata MATHIEU JORON1*, IAN R. WYNNE2, GERARDO LAMAS3 and JAMES MALLET2 1Institut des Sciences de l'Evolution, Universite de MontpellierII, Place E. Bataillon, F-34095 Montpellier, France; 2Department of Biology, University College London, 4, Stephenson Way, London NW1 2HE, UK; 3Departamento de EntomologõÂa, Museo de Historia Natural, Universidad Nacional Mayor de San Marcos, Apartado 14-0434, Lima-14, Peru (*author for correspondence, fax: +31 (0) 71-527-4900; email: [email protected] Inst. Evol. Ecol. Sciences, Univ. Leiden, PO BOX 9516, 2300 RA Leiden, The Netherlands) Received 20 June 2000; accepted 11 December 2000 Co-ordinating editor: C. Rowe Abstract. Polymorphism in aposematic animals and coexistence of multiple mimicry rings within a habitat are not predicted by classical MuÈ llerian mimicry. The butter¯y Heliconius numata Cramer (Lepidoptera: Nymphalidae; Heliconiinae) is both polymorphic and aposematic. The polymor- phism is due to variation at a single locus (or `supergene') which determines colour patterns involved in MuÈ llerian mimicry. We sampled 11 sites in a small area (approx. 60 ´ 30 km) of North- eastern Peru for H. numata and its co-mimics in the genus Melinaea and Athyrtis (Ithomiinae), and examined the role of temporal and spatial heterogeneity in the maintenance of polymorphism. Colour-patterns of Melinaea communities, which constitute the likely `mimetic environment' for H. numata, are dierentiated on a more local scale than morphs of H. numata, but the latter do show a strong and signi®cant response to local selection for colour-pattern. -
Nymphalidae: Ithomiinae)
STUDIES ON THE ECOLOGY AND EVOLUTION OF NEOTROPICAL ITHOMIINE BUTTERFLIES (NYMPHALIDAE: ITHOMIINAE) by GEORGE WILLIAM BECCALONI A thesis submitted for the degree of Doctor ofPhilosophy ofthe University ofLondon October 1995 Biogeography and Conservation Laboratory Centre for Population Biology Department of Entomology Imperial College The Natural History Museum Silwood Park Cromwell Road Ascot London SW7 5BD Berkshire SL5 7PY 2 To my mother, Benjie & Judy in love and gratitude 3 ABSTRACT Two aspects ofthe ecology ofNeotropical ithomiine butterflies (Nymphalidae: Ithomiinae) are discussed: mimicry (Chapters 2, 3) and species richness (Chapters 4, 5). Chapter 2 defines eight mimicry complexes involving ithomiines and other insects found in eastern Ecuador. These complexes are dominated by ithomiine individuals. Hypotheses to explain polymorphism in Batesian and Mullerian mimics are assessed. In Chapter 3, evidence that sympatric ithomiine-dominated mimicry complexes are segregated by microhabitat is reviewed. Data confirm that sympatric complexes are segregated vertically by flight height. Flight height is shown to be positively correlated with larval host-plant height. Host-plant partitioning between species in a butterfly community results in the formation of microhabitat guilds of species, and evidence suggests that mimicry may evolve between species which share a guild, but not between guilds. Models for the evolution of mimicry complexes in sympatry, and for polymorphism and dual sex-limited mimicry in Mullerian mimics, are discussed in the light of these findings. Chapter 4 investigates relationships between species richness offamilies and subfamilies ofNeotropical butterflies and overall butterfly species richness at local and regional scales. A strong positive correlation is demonstrated between ithomiine richness and the species richness of all other butterflies. -
Forest Health Technology Enterprise Team Biological Control of Invasive
Forest Health Technology Enterprise Team TECHNOLOGY TRANSFER Biological Control Biological Control of Invasive Plants in the Eastern United States Roy Van Driesche Bernd Blossey Mark Hoddle Suzanne Lyon Richard Reardon Forest Health Technology Enterprise Team—Morgantown, West Virginia United States Forest FHTET-2002-04 Department of Service August 2002 Agriculture BIOLOGICAL CONTROL OF INVASIVE PLANTS IN THE EASTERN UNITED STATES BIOLOGICAL CONTROL OF INVASIVE PLANTS IN THE EASTERN UNITED STATES Technical Coordinators Roy Van Driesche and Suzanne Lyon Department of Entomology, University of Massachusets, Amherst, MA Bernd Blossey Department of Natural Resources, Cornell University, Ithaca, NY Mark Hoddle Department of Entomology, University of California, Riverside, CA Richard Reardon Forest Health Technology Enterprise Team, USDA, Forest Service, Morgantown, WV USDA Forest Service Publication FHTET-2002-04 ACKNOWLEDGMENTS We thank the authors of the individual chap- We would also like to thank the U.S. Depart- ters for their expertise in reviewing and summariz- ment of Agriculture–Forest Service, Forest Health ing the literature and providing current information Technology Enterprise Team, Morgantown, West on biological control of the major invasive plants in Virginia, for providing funding for the preparation the Eastern United States. and printing of this publication. G. Keith Douce, David Moorhead, and Charles Additional copies of this publication can be or- Bargeron of the Bugwood Network, University of dered from the Bulletin Distribution Center, Uni- Georgia (Tifton, Ga.), managed and digitized the pho- versity of Massachusetts, Amherst, MA 01003, (413) tographs and illustrations used in this publication and 545-2717; or Mark Hoddle, Department of Entomol- produced the CD-ROM accompanying this book. -
Ecological Divergence and Speciation in Heliconius Cydno and H
Ecological divergence and speciation in Heliconius cydno and H. melpomene by Russell Edward Naisbit A thesis submitted for the degree of Doctor of Philosophy of the University of London September 2001 Department of Biology University College London To my family, for their support and encouragement throughout this crazy endeavour 2 “It is hardly an exaggeration to say, that whilst reading and reflecting on the various facts given in this Memoir, we feel to be as near witnesses, as we can ever hope to be, of the creation of a new species on this earth.” Charles Darwin, Natural History Review: Quarterly Journal of Biological Science, 1863. From a review of “Contributions to an Insect Fauna of the Amazon Valley,” in which Henry Walter Bates gave an adaptive explanation for mimicry in Amazonian butterflies and argued that variation in mimicry might cause speciation 3 Abstract We are in the midst of a renaissance in speciation research. There is a return to Darwin’s belief in the role of natural selection in driving speciation, after a lengthy focus on geographic isolation and hybrid sterility. Here I describe the ecological, behavioural, and genetic bases of speciation in Heliconius cydno and Heliconius melpomene (Lepidoptera: Nymphalidae). The two species are sympatric in tropical rainforest across most of Central America and the foothills of the Andes. Ecological differentiation allows coexistence of these sister species despite rare hybridisation. Divergence in microhabitat and larval host plant use has reduced both the potential for gene flow and for competition. In Panama H. cydno uses most Passiflora species in closed canopy forest, whilst H.