Reaction Kinetics of Versatile Peroxidase for the Degradation of Lignin Compounds
Total Page:16
File Type:pdf, Size:1020Kb
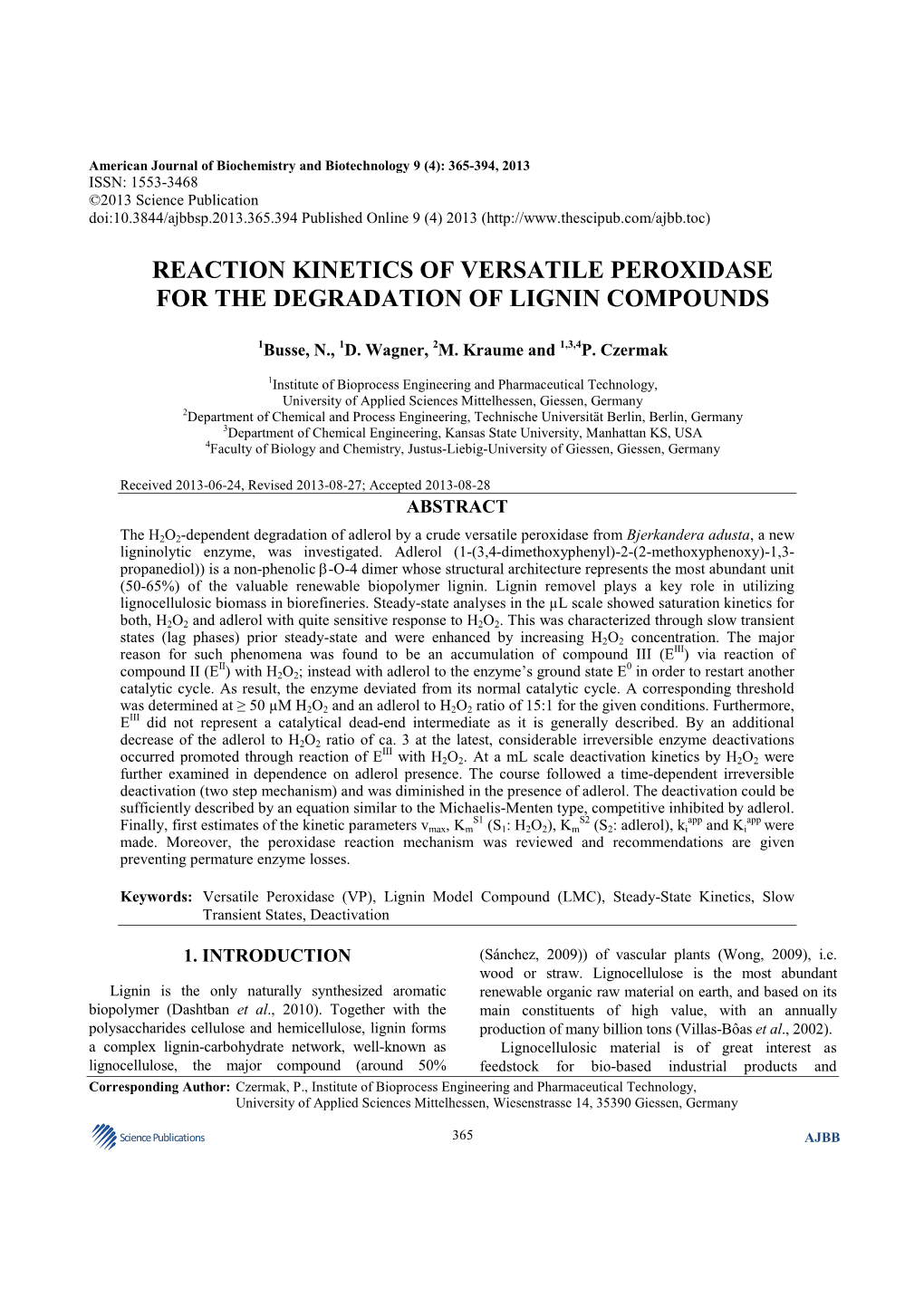
Load more
Recommended publications
-
The Role of Protein Crystallography in Defining the Mechanisms of Biogenesis and Catalysis in Copper Amine Oxidase
Int. J. Mol. Sci. 2012, 13, 5375-5405; doi:10.3390/ijms13055375 OPEN ACCESS International Journal of Molecular Sciences ISSN 1422-0067 www.mdpi.com/journal/ijms Review The Role of Protein Crystallography in Defining the Mechanisms of Biogenesis and Catalysis in Copper Amine Oxidase Valerie J. Klema and Carrie M. Wilmot * Department of Biochemistry, Molecular Biology, and Biophysics, University of Minnesota, 321 Church St. SE, Minneapolis, MN 55455, USA; E-Mail: [email protected] * Author to whom correspondence should be addressed; E-Mail: [email protected]; Tel.: +1-612-624-2406; Fax: +1-612-624-5121. Received: 6 April 2012; in revised form: 22 April 2012 / Accepted: 26 April 2012 / Published: 3 May 2012 Abstract: Copper amine oxidases (CAOs) are a ubiquitous group of enzymes that catalyze the conversion of primary amines to aldehydes coupled to the reduction of O2 to H2O2. These enzymes utilize a wide range of substrates from methylamine to polypeptides. Changes in CAO activity are correlated with a variety of human diseases, including diabetes mellitus, Alzheimer’s disease, and inflammatory disorders. CAOs contain a cofactor, 2,4,5-trihydroxyphenylalanine quinone (TPQ), that is required for catalytic activity and synthesized through the post-translational modification of a tyrosine residue within the CAO polypeptide. TPQ generation is a self-processing event only requiring the addition of oxygen and Cu(II) to the apoCAO. Thus, the CAO active site supports two very different reactions: TPQ synthesis, and the two electron oxidation of primary amines. Crystal structures are available from bacterial through to human sources, and have given insight into substrate preference, stereospecificity, and structural changes during biogenesis and catalysis. -
Measuring Phenol Oxidase and Peroxidase Activities with Pyrogallol, L-DOPA, and ABTS: Effect of Assay Conditions and Soil Type
UC Irvine UC Irvine Previously Published Works Title Measuring phenol oxidase and peroxidase activities with pyrogallol, l-DOPA, and ABTS: Effect of assay conditions and soil type Permalink https://escholarship.org/uc/item/3nw4m85r Authors Bach, CE Warnock, DD Van Horn, DJ et al. Publication Date 2013-12-01 DOI 10.1016/j.soilbio.2013.08.022 Peer reviewed eScholarship.org Powered by the California Digital Library University of California SBB5589_grabs ■ 10 September 2013 ■ 1/1 Soil Biology & Biochemistry xxx (2013) 1 Contents lists available at ScienceDirect Soil Biology & Biochemistry journal homepage: www.elsevier.com/locate/soilbio 1 2 3 4 Highlights 5 6 We tested whether pH and redox potential affect three common oxidase substrates. 7 Pyrogallol and ABTS were useless under alkaline conditions for different reasons. 8 L-DOPA appears to be stable for use across a broad range of pH. 9 Autoclaved and combusted soils cannot be used as negative controls. 10 Current “oxidase” methods measure a soil property more so than enzyme activity. 11 12 0038-0717/$ e see front matter Ó 2013 Published by Elsevier Ltd. http://dx.doi.org/10.1016/j.soilbio.2013.08.022 Please cite this article in press as: Bach, C.E., et al., Measuring phenol oxidase and peroxidase activities with pyrogallol, L-DOPA, and ABTS: Effect of assay conditions and soil type, Soil Biology & Biochemistry (2013), http://dx.doi.org/10.1016/j.soilbio.2013.08.022 SBB5589_proof ■ 10 September 2013 ■ 1/9 Soil Biology & Biochemistry xxx (2013) 1e9 Contents lists available at ScienceDirect Soil Biology & Biochemistry journal homepage: www.elsevier.com/locate/soilbio 1 56 2 Measuring phenol oxidase and peroxidase activities with pyrogallol, 57 3 58 4 L-DOPA, and ABTS: Effect of assay conditions and soil type 59 5 60 6 a b b c 61 Q5 Christopher E. -
Oxidative Power: Tools for Assessing LPMO Activity on Cellulose
biomolecules Review Oxidative Power: Tools for Assessing LPMO Activity on Cellulose Federica Calderaro 1,2,* , Loes E. Bevers 1 and Marco A. van den Berg 1 1 DSM Biotechnology Center, 2613 AX Delft, The Netherlands; [email protected] (L.E.B.); [email protected] (M.A.v.d.B.) 2 Molecular Enzymolog y Group, University of Groningen, Nijenborgh 4, 9747 AG Groningen, The Netherlands * Correspondence: [email protected]; Tel.: +31-6-36028569 Abstract: Lytic polysaccharide monooxygenases (LPMOs) have sparked a lot of research regarding their fascinating mode-of-action. Particularly, their boosting effect on top of the well-known cel- lulolytic enzymes in lignocellulosic hydrolysis makes them industrially relevant targets. As more characteristics of LPMO and its key role have been elucidated, the need for fast and reliable methods to assess its activity have become clear. Several aspects such as its co-substrates, electron donors, inhibiting factors, and the inhomogeneity of lignocellulose had to be considered during experimental design and data interpretation, as they can impact and often hamper outcomes. This review provides an overview of the currently available methods to measure LPMO activity, including their potential and limitations, and it is illustrated with practical examples. Keywords: lytic polysaccharide monooxygenase; enzyme assay; cellulose; lignocellulosic biomass; chromatography; enzyme kinetics Citation: Calderaro, F.; Bevers, L.E.; van den Berg, M.A. Oxidative Power: Tools for Assessing LPMO Activity 1. Introduction on Cellulose. Biomolecules 2021, 11, Until recently, the enzymatic conversion of recalcitrant polysaccharidic materials such 1098. https://doi.org/10.3390/ as chitin or cellulose was thought to solely depend on the activity of hydrolytic enzymes. -
Deconstruction of Lignin: from Enzymes to Microorganisms
molecules Review Deconstruction of Lignin: From Enzymes to Microorganisms Jéssica P. Silva 1, Alonso R. P. Ticona 1 , Pedro R. V. Hamann 1, Betania F. Quirino 2 and Eliane F. Noronha 1,* 1 Enzymology Laboratory, Cell Biology Department, University of Brasilia, 70910-900 Brasília, Brazil; [email protected] (J.P.S.); [email protected] (A.R.P.T.); [email protected] (P.R.V.H.) 2 Genetics and Biotechnology Laboratory, Embrapa-Agroenergy, 70770-901 Brasília, Brazil; [email protected] * Correspondence: [email protected]; Tel.: +55-61-3307-2152 Abstract: Lignocellulosic residues are low-cost abundant feedstocks that can be used for industrial applications. However, their recalcitrance currently makes lignocellulose use limited. In natural environments, microbial communities can completely deconstruct lignocellulose by synergistic action of a set of enzymes and proteins. Microbial degradation of lignin by fungi, important lignin degraders in nature, has been intensively studied. More recently, bacteria have also been described as able to break down lignin, and to have a central role in recycling this plant polymer. Nevertheless, bacterial deconstruction of lignin has not been fully elucidated yet. Direct analysis of environmental samples using metagenomics, metatranscriptomics, and metaproteomics approaches is a powerful strategy to describe/discover enzymes, metabolic pathways, and microorganisms involved in lignin breakdown. Indeed, the use of these complementary techniques leads to a better understanding of the composition, function, and dynamics of microbial communities involved in lignin deconstruction. We focus on omics approaches and their contribution to the discovery of new enzymes and reactions that impact the development of lignin-based bioprocesses. -
Insights Into the Oxidative Degradation of Cellulose by a Copper Metalloenzyme That Exploits Biomass Components
Insights into the oxidative degradation of cellulose by a copper metalloenzyme that exploits biomass components R. Jason Quinlana,1, Matt D. Sweeneya,1, Leila Lo Leggiob, Harm Ottenb, Jens-Christian N. Poulsenb, Katja Salomon Johansenc,2, Kristian B. R. M. Kroghc, Christian Isak Jørgensenc, Morten Tovborgc, Annika Anthonsenc, Theodora Tryfonad, Clive P. Walterc, Paul Dupreed, Feng Xua, Gideon J. Daviese, and Paul H. Waltone aNovozymes, Inc., Davis, CA 95618; bDepartment of Chemistry, University of Copenhagen, 2100 Copenhagen Ø, Denmark; cNovozymes A/S, DK-2880 Bagsværd, Denmark; dDepartment of Biochemistry, School of Biological Sciences, University of Cambridge, Cambridge CB2 1QW, United Kingdom; and eDepartment of Chemistry, University of York, Heslington, York YO10 5DD, United Kingdom Edited* by Diter von Wettstein, Washington State University, Pullman, WA, and approved August 2, 2011 (received for review April 13, 2011) The enzymatic degradation of recalcitrant plant biomass is one lysis. From this work, it was suggested that GH61s act directly on of the key industrial challenges of the 21st century. Accordingly, cellulose rendering it more accessible to traditional cellulase there is a continuing drive to discover new routes to promote action (11). Moreover, recent genomic sequencing of the brown polysaccharide degradation. Perhaps the most promising approach rot fungi Postia placenta showed a number of GH61 genes in this involves the application of “cellulase-enhancing factors,” such as organism (13–15), indicating the widespread nature of this those from the glycoside hydrolase (CAZy) GH61 family. Here we family of enzymes in cellulose degradation. As such, GH61s show that GH61 enzymes are a unique family of copper-depen- likely hold major potential for industrial decomposition of dent oxidases. -
Evaluation of Oxidative Enzymes in Leaf Tissue from Intact Cotton Plants Exposed to Different Oxygen Concentrations
EVALUATION OF OXIDATIVE ENZYMES IN LEAF TISSUE FROM INTACT COTTON PLANTS EXPOSED TO DIFFERENT OXYGEN CONCENTRATIONS by Joyce Geraldine Foster Dissertation submitted to the Graduate Faculty of the Virginia Polytechnic Institute and State University in partial fulfillment of the requirements for the degree of DOCTOR OF PHILOSOPHY in Biochemistry and Nutrition APPROVED: J. L. Hess, Chairman L. B. Barnett R. D. Brown, Jr. 't. M. Grego2'y L. D. Moore R. R. Schmidt March,1979 Blacksburg, Virginia ACKNOWLEDGMENTS My deepest appreciation is extended to my advisor, Dr. John L. Hess, whose assistance, optimism, and con- stant encouragement during this project made even the darkest hours seem bright. A heartfelt thank you goes to my committee members, Dr. L. B. Barnett, Dr. R. D. Brown, Jr., Dr. E. M. Gregory, Dr. L. D. Moore, and Dr. R. R. Schmidt, for their support and guida~ce throughout the degree program and especially during Dr. Hess' sabba- tical. I especially wish to acknowledge for arranging extended use of growth chambers and instru- ments for monitoring environmental conditions and tissue properties and particularly for his invaluable technical advice. Special thanks are express2d to Dr. E. M. Gregory for his constant assistance with experimental design 2nd maintenance of equipment. The follJwing people who so generously made their instruments available during the course of this project are also grc. l:efully acknowledged: Dr. E. M. Gregory, I Dr. R. D. Brown, Jr• I I and Dr. R. R. Schmidt. I am also grateful to ii , and the personnel of the Virginia Polytechnic Institute and State University soils testing lab for their assistance in the identification of optimal culture condi- tions for cotton in controlled environments; to and personnel of the Virginia Polytechnic Institute and State University statistics consulting center for as- sistance with statistical analysis of data; to for competent technical assistance; to , and all the fellows in the department for moving untold numbers of compressed gas cylinders; and to and for typing this dissertation. -
Oxidative Degradation of Non-Phenolic Lignin During Lipid Peroxidation by Fungal Manganese Peroxidase
FEBS Letters 354 (1994) 297-300 FEBS 14759 Oxidative degradation of non-phenolic lignin during lipid peroxidation by fungal manganese peroxidase Wuli Bao, Yaichi Fukushima, Kenneth A. Jensen Jr., Mark A. Moen, Kenneth E. Hammel* USDA Forest Products Laboratory, Madison, WI 53705, USA Received 3 October 1994 Abstract A non-phenolic lignin model dimer, 1-(4-ethoxy-3-methoxyphenyl)-2-phenoxypropane-l ,3-diol, was oxidized by a lipid peroxidation system that consisted of a fungal manganese peroxidase, Mn(II), and unsaturated fatty acid esters. The reaction products included 1-(4-ethoxy-3- methoxyphenyl)-1-oxo-2-phenoxy-3-hydroxypropane and 1-(4-ethoxy-3-methoxyphenyl)- 1-oxo-3-hydroxypropane, indicating that substrate oxida- tion occurred via benzylic hydrogen abstraction. The peroxidation system depolymerized both exhaustively methylated (non-phenolic) and unmeth- ylated (phenolic) synthetic lignins efficiently. It may therefore enable white-rot fungi to accomplish the initial delignification of wood. Key words: Manganese peroxidase; Lipid peroxidation; Ligninolysis; Non-phenolic lignin; White-rot fungus 1. Introduction 2. Materials and methods White-rot fungi have evolved unique ligninolytic mecha- 2.1. Reagents l-(4-Ethoxy-3-methoxyphenyl)-2-phenoxypropane-l,3-diol (I) [12] nisms, which enable them to play an essential role in terrestrial 1-(4-ethoxy-3-methoxyphenyl)-l-oxo-2-phenoxy-3-hydroxypropane ecosystems by degrading and recycling lignified biomass. The (II) [13], and l-(4-ethoxy-3-methoxyphenyl)-l-oxo-3-hydroxypropane chemical recalcitrance, random structure, and large size of lig- (III) [14] were prepared by the indicated methods.I labeled with 14C at Cl (0.066 mCi· mmol-1) was prepared in the same manner as the unla- nin require that these mechanisms be oxidative, non-specific, 14 beled compound, using [1- C]acetic acid as the labeled precursor. -
Bioresources.Com
PEER-REVIEWED ARTICLE bioresources.com HYPERPRODUCTIVITY OF EXTRACELLULAR ENZYMES FROM INDIGENOUS WHITE ROT FUNGI (P. chrysosporium IBL-03) BY UTILIZING AGRO-WASTES Muhammad Asgher, Nadeem Ahmed, and Hafiz Muhammad Nasir Iqbal* An indigenous locally isolated white rot fungal strain Phanerochaete chrysosporium IBL-03 was investigated for the hyper-production of ligninolytic enzymes from different agro-industrial wastes including wheat straw, rice straw, banana stalks, corncobs, corn stover, and sugarcane bagasse as substrate material in still culture fermentation technique. Screening experiments were performed at 30oC from 1 to 10 days and maximum enzyme activities were recorded after the 5th day of incubation on banana stalk. P. chrysosporium IBL-03 produced highest activities of lignin peroxidase (LiP) and manganese peroxidase (MnP) but no laccase activity was detected in any fermented culture media. Production of ligninolytic enzymes was substantially enhanced through the optimization process. When banana stalk at 66.6 % moisture level and pH 4.5 was inoculated with 5mL spore suspension of P. chrysosporium IBL-03 at 35oC in the presence of molasses (1%) as carbon source, ammonium sulfate (0.2%) as nitrogen supplement, (1%) Tween-80 (0.3 mL) as surfactant and mediators (MnSO4 and veratryl alcohol) enhanced the LiP and MnP production up to 1040 and 965 (U/mL), respectively. Keywords: Phanerochaete chrysosporium IBL-03; Extracellular enzymes; Agro-wastes; SSF; Optimization Contact information: Department of Chemistry and Biochemistry, University of Agriculture, Faisalabad Pakistan; * Corresponding Author: Telephone# +92-307-6715243 E-mail: [email protected] INTRODUCTION White rot fungi (WRF) are among the most robust micro-organisms, having the ability to degrade the major components of lignocellulosic sources, cellulose, hemicelluloses, and lignin as available hydrolyzable nutrients efficiently through their nonspecific and non stereo-selective extracellular enzyme system (Papinutti and Forchiassin 2007; Ruhl et al. -
Kinetic Approaches to Measuring Peroxiredoxin Reactivity
Mol. Cells 2016; 39(1): 26-30 http://dx.doi.org/10.14348/molcells.2016.2325 Molecules and Cells http://molcells.org Established in 1990 Kinetic Approaches to Measuring Peroxiredoxin Reactivity Christine C. Winterbourn*, and Alexander V. Peskin Peroxiredoxins are ubiquitous thiol proteins that catalyse demonstrated surprisingly low reactivity with thiol reagents such the breakdown of peroxides and regulate redox activity in as iodoacetamide and other oxidants such as chloramines the cell. Kinetic analysis of their reactions is required in (Peskin et al., 2007) and it is clear that the low pKa of the active order to identify substrate preferences, to understand how site thiol is insufficient to confer the high peroxide reactivity. In molecular structure affects activity and to establish their fact, typical low molecular weight and protein thiolates react -1 -1 physiological functions. Various approaches can be taken, with H2O2 with a rate constant of 20 M s whereas values of including the measurement of rates of individual steps in Prxs are 105-106 fold higher (Winterbourn and Hampton, 2008). the reaction pathway by stopped flow or competitive kinet- An elegant series of structural and mutational studies (Hall et al., ics, classical enzymatic analysis and measurement of pe- 2010; Nakamura et al., 2010; Nagy et al., 2011) have shown roxidase activity. Each methodology has its strengths and that to get sufficient rate enhancement, it is necessary to acti- they can often give complementary information. However, vate the peroxide. As discussed in detail elsewhere (Hall et al., it is important to understand the experimental conditions 2010; 2011), this involves formation of a transition state in of the assay so as to interpret correctly what parameter is which hydrogen bonding of the peroxide to conserved Arg and being measured. -
Prokaryotic Origins of the Non-Animal Peroxidase Superfamily and Organelle-Mediated Transmission to Eukaryotes
View metadata, citation and similar papers at core.ac.uk brought to you by CORE provided by Elsevier - Publisher Connector Genomics 89 (2007) 567–579 www.elsevier.com/locate/ygeno Prokaryotic origins of the non-animal peroxidase superfamily and organelle-mediated transmission to eukaryotes Filippo Passardi a, Nenad Bakalovic a, Felipe Karam Teixeira b, Marcia Margis-Pinheiro b,c, ⁎ Claude Penel a, Christophe Dunand a, a Laboratory of Plant Physiology, University of Geneva, Quai Ernest-Ansermet 30, CH-1211 Geneva 4, Switzerland b Department of Genetics, Institute of Biology, Federal University of Rio de Janeiro, Rio de Janeiro, Brazil c Department of Genetics, Federal University of Rio Grande do Sul, Rio Grande do Sul, Brazil Received 16 June 2006; accepted 18 January 2007 Available online 13 March 2007 Abstract Members of the superfamily of plant, fungal, and bacterial peroxidases are known to be present in a wide variety of living organisms. Extensive searching within sequencing projects identified organisms containing sequences of this superfamily. Class I peroxidases, cytochrome c peroxidase (CcP), ascorbate peroxidase (APx), and catalase peroxidase (CP), are known to be present in bacteria, fungi, and plants, but have now been found in various protists. CcP sequences were detected in most mitochondria-possessing organisms except for green plants, which possess only ascorbate peroxidases. APx sequences had previously been observed only in green plants but were also found in chloroplastic protists, which acquired chloroplasts by secondary endosymbiosis. CP sequences that are known to be present in prokaryotes and in Ascomycetes were also detected in some Basidiomycetes and occasionally in some protists. -
No Temperature Acclimation of Soil Extracellular Enzymes to Experimental Warming in an Alpine Grassland Ecosystem on the Tibetan Plateau
Biogeochemistry DOI 10.1007/s10533-013-9844-2 No temperature acclimation of soil extracellular enzymes to experimental warming in an alpine grassland ecosystem on the Tibetan Plateau Xin Jing • Yonghui Wang • Haegeun Chung • Zhaorong Mi • Shiping Wang • Hui Zeng • Jin-Sheng He Received: 12 September 2012 / Accepted: 28 March 2013 Ó Springer Science+Business Media Dordrecht 2013 Abstract Alpine grassland soils store large amounts Tibetan Plateau. A free air-temperature enhancement of soil organic carbon (SOC) and are susceptible to system was set up in May 2006. We measured soil rising air temperature. Soil extracellular enzymes microbial biomass, nutrient availability and the activity catalyze the rate-limiting step in SOC decomposition of five extracellular enzymes in 2009 and 2010. The Q10 and their catalysis, production and degradation rates are of each enzyme was calculated using a simple first-order regulated by temperature. Therefore, the responses of exponential equation. We found that warming had no these enzymes to warming could have a profound significant effects on soil microbial biomass C, the labile impact on carbon cycling in the alpine grassland C or N content, or nutrient availability. Significant ecosystems. This study was conducted to measure the differences in the activity of most extracellular enzymes responses of soil extracellular enzyme activity and among sampling dates were found, with typically higher temperature sensitivity (Q10) to experimental warming enzyme activity during the warm period of the year. The in samples from an alpine grassland ecosystem on the effects of warming on the activity of the five extracel- lular enzymes at 20 °C were not significant. -
Bioresources.Com
PEER-REVIEWED REVIEW ARTICLE bioresources.com BIOLOGICAL PRETREATMENT OF LIGNOCELLULOSES WITH WHITE-ROT FUNGI AND ITS APPLICATIONS: A REVIEW a,b c, d Isroi, Ria Millati, * Siti Syamsiah, Claes Niklasson,b Muhammad Nur Cahyanto,c f Knut Lundquist,e and Mohammad J. Taherzadeh Lignocellulosic carbohydrates, i.e. cellulose and hemicellulose, have abundant potential as feedstock for production of biofuels and chemicals. However, these carbohydrates are generally infiltrated by lignin. Breakdown of the lignin barrier will alter lignocelluloses structures and make the carbohydrates accessible for more efficient bioconversion. White-rot fungi produce ligninolytic enzymes (lignin peroxidase, manganese peroxidase, and laccase) and efficiently mineralise lignin into CO2 and H2O. Biological pretreatment of lignocelluloses using white-rot fungi has been used for decades for ruminant feed, enzymatic hydrolysis, and biopulping. Application of white-rot fungi capabilities can offer environmentally friendly processes for utilising lignocelluloses over physical or chemical pretreatment. This paper reviews white-rot fungi, ligninolytic enzymes, the effect of biological pretreatment on biomass characteristics, and factors affecting biological pretreatment. Application of biological pretreatment for enzymatic hydrolysis, biofuels (bioethanol, biogas and pyrolysis), biopulping, biobleaching, animal feed, and enzymes production are also discussed. Keywords: Biological pretreatment; White-rot fungi; Lignocellulose; Solid-state fermentation; Lignin peroxidase;