Exploring Mars with Returned Samples
Total Page:16
File Type:pdf, Size:1020Kb
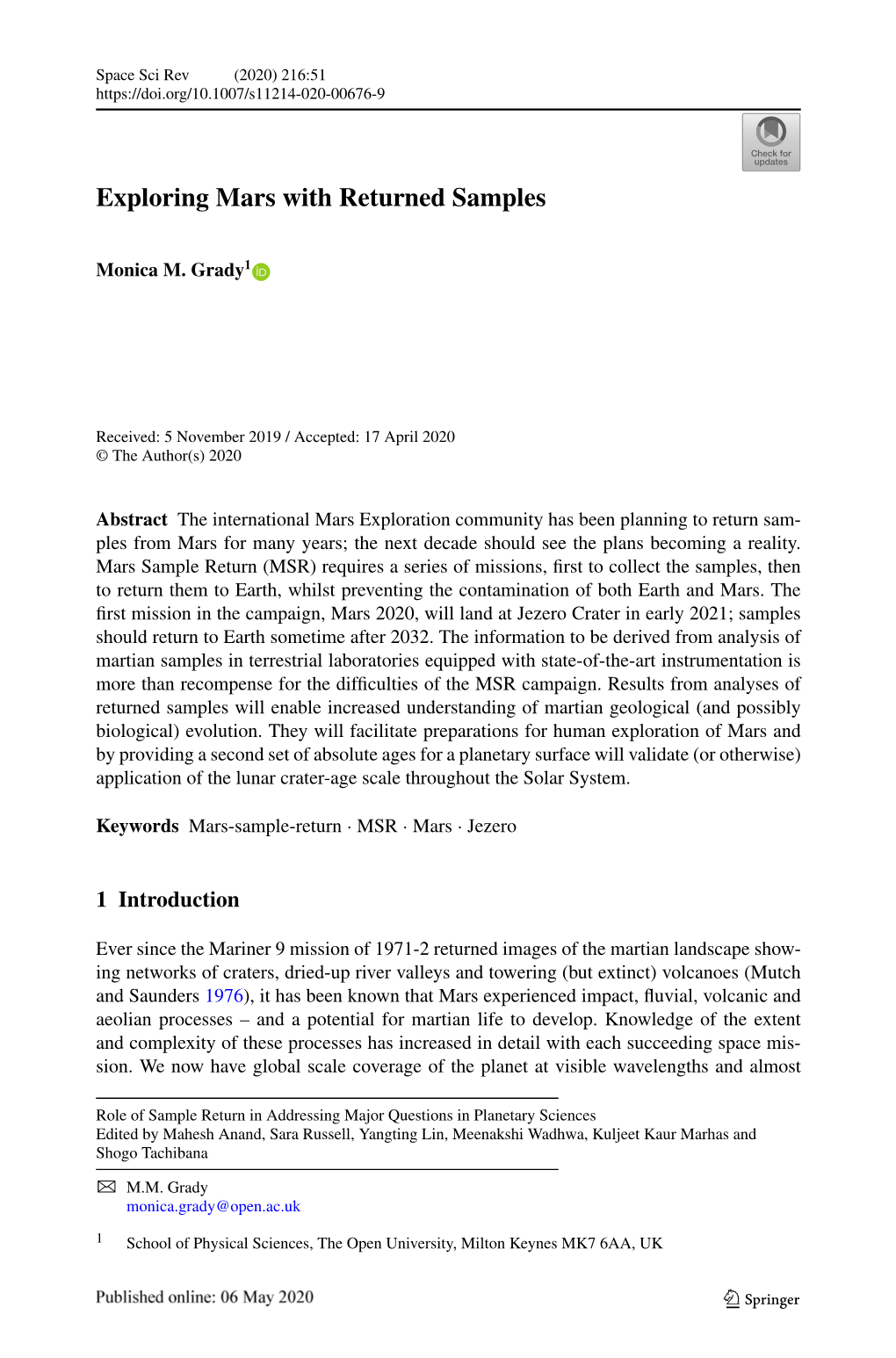
Load more
Recommended publications
-
GSA ROCKY MOUNTAIN/CORDILLERAN JOINT SECTION MEETING 15–17 May Double Tree by Hilton Hotel and Conference Center, Flagstaff, Arizona, USA
Volume 50, Number 5 GSA ROCKY MOUNTAIN/CORDILLERAN JOINT SECTION MEETING 15–17 May Double Tree by Hilton Hotel and Conference Center, Flagstaff, Arizona, USA www.geosociety.org/rm-mtg Sunset Crater is a cinder cone located north of Flagstaff, Arizona, USA. Program 05-RM-cvr.indd 1 2/27/2018 4:17:06 PM Program Joint Meeting Rocky Mountain Section, 70th Meeting Cordilleran Section, 114th Meeting Flagstaff, Arizona, USA 15–17 May 2018 2018 Meeting Committee General Chair . Paul Umhoefer Rocky Mountain Co-Chair . Dennis Newell Technical Program Co-Chairs . Nancy Riggs, Ryan Crow, David Elliott Field Trip Co-Chairs . Mike Smith, Steven Semken Short Courses, Student Volunteer . Lisa Skinner Exhibits, Sponsorship . Stephen Reynolds GSA Rocky Mountain Section Officers for 2018–2019 Chair . Janet Dewey Vice Chair . Kevin Mahan Past Chair . Amy Ellwein Secretary/Treasurer . Shannon Mahan GSA Cordilleran Section Officers for 2018–2019 Chair . Susan Cashman Vice Chair . Michael Wells Past Chair . Kathleen Surpless Secretary/Treasurer . Calvin Barnes Sponors We thank our sponsors below for their generous support. School of Earth and Space Exploration - Arizona State University College of Engineering, Forestry, and Natural Sciences University of Arizona Geosciences (Arizona LaserChron Laboratory - ALC, Arizona Radiogenic Helium Dating Lab - ARHDL) School of Earth Sciences & Environmental Sustainability - Northern Arizona University Arizona Geological Survey - sponsorship of the banquet Prof . Stephen J Reynolds, author of Exploring Geology, Exploring Earth Science, and Exploring Physical Geography - sponsorship of the banquet NOTICE By registering for this meeting, you have acknowledged that you have read and will comply with the GSA Code of Conduct for Events (full code of conduct listed on page 31) . -
The Geological History of Nili Patera, Mars 10.1002/2015JE004795 P
PUBLICATIONS Journal of Geophysical Research: Planets RESEARCH ARTICLE The geological history of Nili Patera, Mars 10.1002/2015JE004795 P. Fawdon1, J. R. Skok2, M. R. Balme1, C. L. Vye-Brown3, D. A. Rothery1, and C. J. Jordan4 Key Points: 1Department of Physical Sciences, The Open University, Walton Hall, Milton Keynes, UK, 2Department of Geology & • A new CTX-scale map details the 3 geological history of Nili Patera Geophysics, Louisiana State University, Baton Rouge, Louisiana, USA, British Geological Survey, Murchison House, 4 • Magmatism includes effusive basalts, Edinburgh, UK, British Geological Survey, Nottingham, UK magmatic intrusion, and ignimbrite(s) • Volcanism and water suggest habitable environments in highland patera caldera Abstract Nili Patera is a 50 km diameter caldera at the center of the Syrtis Major Planum volcanic province. The caldera is unique among Martian volcanic terrains in hosting: (i) evidence of both effusive and explosive Supporting Information: volcanism, (ii) hydrothermal silica, and (iii) compositional diversity from olivine-rich basalts to silica-enriched • Figures S1 and S2 units. We have produced a new geological map using three mosaicked 18 m/pixel Context Camera digital • Map S1 elevation models, supplemented by Compact Remote Imaging Spectrometer for Mars Hyperspectral data. Correspondence to: The map contextualizes these discoveries, formulating a stratigraphy in which Nili Patera formed by trapdoor P. Fawdon, collapse into a volcanotectonic depression. The distinctive bright floor of Nili Patera formed either as part of a [email protected] felsic pluton, exposed during caldera formation, or as remnants of welded ignimbrite(s) associated with caldera formation—both scenarios deriving from melting in the Noachian highland basement. -
Nauny, Philippe É. M. (2019) Interpretation of Biosignatures in Extreme Environments, and Their Potential Impact for the Search for Life on Mars
Nauny, Philippe É. M. (2019) Interpretation of biosignatures in extreme environments, and their potential impact for the search for life on Mars. PhD thesis. http://theses.gla.ac.uk/77875/ Copyright and moral rights for this work are retained by the author A copy can be downloaded for personal non-commercial research or study, without prior permission or charge This work cannot be reproduced or quoted extensively from without first obtaining permission in writing from the author The content must not be changed in any way or sold commercially in any format or medium without the formal permission of the author When referring to this work, full bibliographic details including the author, title, awarding institution and date of the thesis must be given Enlighten: Theses https://theses.gla.ac.uk/ [email protected] Interpretation of biosignatures in extreme environments, and their potential impact for the search for life on Mars Philippe É. M. Nauny Master en Chimie et Biologie Université Louis Pasteur, Strasbourg Submitted in fulfilment of the requirements for the Degree of Doctor of Philosophy School of Geographical and Earth Sciences College of Science and Engineering University of Glasgow Viva: 21st February 2019 Abstract The search for life beyond Earth, and on Mars in particular, is one of the key points of astrobiology research. However, space missions are expensive and time-consuming. Simu- lating such missions at analogue sites on Earth can thus save time and money whilst working in natural settings. This thesis presents three studies of biosignatures from three different martian analogues. These biosignatures were linked — when possible — to environmental parameters observed at their sampling sites. -
High-Precision Topographic Map of the Mars 2020 Landing Site As Part of the Mc-13E Syrtis Major Quadrangle Digital Terrain Model
52nd Lunar and Planetary Science Conference 2021 (LPI Contrib. No. 2548) 2509.pdf HIGH-PRECISION TOPOGRAPHIC MAP OF THE MARS 2020 LANDING SITE AS PART OF THE MC-13E SYRTIS MAJOR QUADRANGLE DIGITAL TERRAIN MODEL. A. Neesemann1, S.H.G. Walter1, C. Gross1, R. Jaumann1, K. Gwin- ner2, G.G. Michael1, B.P. Schreiner,1 W. Zuschneid1, D. Neu1, H. Balthasar1, C. Rabethge1, C. Riedel1, E. Kersten2, D. Tirsch2 1Freie Universität Berlin, Institute of Geological Sciences, Planetary Sciences and Remote Sensing. Malteserstr. 74-100, 12249 Berlin, Germany ([email protected]), 2German Aerospace Center (DLR) Berlin, Institute of Planetary Research, Ruther- fordstr.2, 12489 Berlin, Germany 67.5°E 90°E Introduction 30°N 30°N For more than 17 years, the High Resolution Stereo Camera (HRSC) [1] onboard Mars Express (MEx) has acquired image data of the Red Planet on a global scale and at high resolution. 3164 E Although outmatched in spatial resolution by the Context A S S O F Camera (CTX) [2], the High Resolution Imaging Science I L I Experiment (HiRISE) [3] and more recently by the Colour and (m) GMM3 Mars Areoid above Height N Stereo Surface Imaging System (CaSSIS) [4], providing essenti- al and high quality data necessary for detailed geological and geomorphological studies, the unique capabilities of the HRSC Fig. 3 lie in its virtually simultaneous coverage of large areas by ve panchromatic and four narrow band lters (RGB and IR) at I S I D I S dierent phase angles. Digital Terrain Models (DTMs) P L A N I T I A resulting from high-precision 3D point accuracy and density S Y usually have a grid space of 50 m with at least one 3D point per R T I grid cell and are subsequently tied to the Mars Orbiter Laser S M Altimeter (MOLA) global reference system [5]. -
Bulletin of the Mineralogical Society of Southern California
Bulletin of the Mineralogical Society of Southern California Volume 90 Number 11 - November, 2017 The 950th meeting of the Mineralogical Society of Southern California With Knowledge Comes Appreciation November 10th, 2017 at 7:30 P.M. Pasadena City College Geology Department, E-Building, Room 110 1570 E Colorado Blvd., Pasadena Program: A Visit to The Pasadena City College Mineral Museum, By Bruce Carter *Note the room number above is 110, not 220 In this Issue: TITLE Page Program: A Visit to The Pasadena City College Mineral Museum By: Bruce Carter 2 From the Editor: Linda Elsnau 2 Meanderings from the President: Ann Meister 2 Minutes of the October 13, 2017 Meeting 3 List of Upcoming MSSC Events 6 Update on Burglary at Fallbrook Gem & Mineral Museum 7 Jewel Tunnel Imports Invitation 7 MSSC Banquet Announcement 8 What’s Happing at The Smithsonian: By Denise Nelson 9 Ride Share Listing 9 Calendar of Events 10 Report of Another Theft in The Mineral Hobby! 11 2017 Officers 12 About MSSC 12 Remember: If you change your email or street address, you must let the MSSC Editor and Membership Chair know or we cannot guarantee receipt of future Bulletins About the Program: A Visit to The Pasadena City College Mineral Museum: Hosted by: Bruce Carter Bruce Carter will open the Pasadena City College Mineral Museum for our November 10th meeting. Bruce will also give a talk on the history and the contents of the PCC Mineral Museum. We will meet in room E-120, which is located directly below our usual meeting room. -
Aas 19-233 Mars Reconnaissance Orbiter Maneuver Plan for Mars 2020 Entry, Descent, and Landing Support and Beyond
(Preprint) AAS 19-233 MARS RECONNAISSANCE ORBITER MANEUVER PLAN FOR MARS 2020 ENTRY, DESCENT, AND LANDING SUPPORT AND BEYOND Sean V. Wagner∗ and Premkumar R. Menony Jet Propulsion Laboratory, California Institute of Technology The Mars Reconnaissance Orbiter (MRO) spacecraft continues to perform valu- able science observations at Mars, provide telecommunication relay for surface assets, and characterize landing sites for future missions. MRO provided primary relay support for the InSight Mission during Entry, Descent, and Landing (EDL) on November 26, 2018. This paper discusses the current maneuver plan to support Mars 2020 EDL and maintain MRO’s orbit for science operations through 2028. INTRODUCTION The Mars Reconnaissance Orbiter (MRO) spacecraft is currently in its fourth extended mission at Mars. The Primary Science Orbit (PSO) for MRO operations is a 252 km × 317 km altitude, sun-synchronous orbit with the periapsis frozen over the south pole and the ascending node at 3:00 PM Local Mean Solar Time (LMST). In addition to making science observations at Mars, MRO is expected to continue providing relay support for surface assets. For telecommunication and imaging support during the InSight Mission’s Entry, Descent and Landing (EDL) sequence on November 26, 2018, MRO had moved its orbit to around 2:52 PM LMST.1 To support Mars 2020 for its scheduled landing on February 18, 2021, MRO has been requested to transition to a 3:15 PM LMST by the time of EDL. MRO may also support the ExoMars 2020 Mission which is planned to land on Mars shortly thereafter. This paper will discuss the current maneuver plan to satisfy Mars 2020 EDL support requirements. -
Mars Surveyor 2001 Landing Site Workshop
_=_MARS SURVEYOR 2001 LANDING SITE WORKSHOP NASA-AMES RESEARCH CENTER MOFFETT FIELD, CALIFORNIA JANUARY 26-27, 1998 mi MARS SURVEYOR 2001 LANDING SITE WORKSHOP Edited and Prepared by: Virginia C. Gulick Steering Committee: Steve Saunders Geoff Briggs Chris McKay Mike Duke David Paige Mike Carr Patricia Rogers David Crown Jim Zimbleman Organizers: Steve Saunders (MEDSO) and Geoff Briggs (ARC) Organizing Committee: Aaron Zent Ginny Gulick Jim Rice Steve Clifford Held at NASA Ames Research Center January 26-27, 1998 nb t* 'ql ,i MARS SURVEYOR 2001 LANDING SITE WORKSHOP PROGRAM MONDAY 26TH: MORNING Moderator: Geoff Briggs 8:30 INTRODUCTION AND WELCOME. G. Briggs* 8:45 MSP '01 STATUS/SURVEYOR SITE SELECTION PROCESS. D. McCleese* 9:15 THE ATHENA MARS ROVER SCIENCE PAYLOAD. S.W. Squyres*, J.F. Bell III, M. Cart, P. Christensen, D. Des Marais, T. Economou, S. Gorevan, G. KlingelhOfer, L Haskin, K. Herkenhoff, A. Knoll, J.M. Knudsen, M. MaIin, H. McSween, R. Morris, R. Rieder, M. Sims, L Soderblom, H. Wanke, T. Wdowiak. 9:45 A MISSION MODEL FOR THE 2001 MARS ROVER/ATHENA PAYLOAD. R. E. Arvidson*, C. S. Niebur, and J. Bowman. 10:15 MGS RESULTS. M. Carr* 10:45 SELECTION AND ASSESSMENT OF PREDICTIONS OF THE MARS PATHFINDER LANDING SITE. M.P. Golombek*, H. J. Moore, A. F. C. Haldemann, R. A. Cook, T. J. Parker, and J. T. SchofieId. 11:15 USEFUL RADAR DATA FOR MARS 2001 LANDING SITE SELECTION. A. F. C Haldemann*, M. A. Slade, R. F. Jurgens 11:45 LANDING SITE MISSION IMPACTS. D. Spencer* and the Mars Surveyor 2001 Project, Mission Design & Navigation Team. -
Lithosphere.Gsapubs.Org on November 28, 2012
Downloaded from lithosphere.gsapubs.org on November 28, 2012 Lithosphere An episodic slab-rollback model for the origin of the Tharsis rise on Mars: Implications for initiation of local plate subduction and final unification of a kinematically linked global plate-tectonic network on Earth An Yin Lithosphere 2012;4;553-593 doi: 10.1130/L195.1 Email alerting services click www.gsapubs.org/cgi/alerts to receive free e-mail alerts when new articles cite this article Subscribe click www.gsapubs.org/subscriptions/ to subscribe to Lithosphere Permission request click http://www.geosociety.org/pubs/copyrt.htm#gsa to contact GSA Copyright not claimed on content prepared wholly by U.S. government employees within scope of their employment. Individual scientists are hereby granted permission, without fees or further requests to GSA, to use a single figure, a single table, and/or a brief paragraph of text in subsequent works and to make unlimited copies of items in GSA's journals for noncommercial use in classrooms to further education and science. This file may not be posted to any Web site, but authors may post the abstracts only of their articles on their own or their organization's Web site providing the posting includes a reference to the article's full citation. GSA provides this and other forums for the presentation of diverse opinions and positions by scientists worldwide, regardless of their race, citizenship, gender, religion, or political viewpoint. Opinions presented in this publication do not reflect official positions of the Society. -
Television Cartography by R
UH ITED STATES DEPARTMENT OF THE INTERIOR GEOLOGICAL SURVEY ·-- - I INTERAGENCY REPORT: ASTROGEOLOGY 58 Television Cartography by R. M. Batson September 1973 Prepared under JPL Contract W0-8122 This report is preliminary and has not been edited or reviewed for conformity with u.s. Geolog ical Survey standards and nomenclature. Prepared by the Geological Survey for the National Aeronautics and Space Administration INTERAGENCY REPORT: ASTROGEOLOGY 58 Television Cartography by R. M. Batson September 1973 Prepared under JPL Contract W0-8122 CONTENTS Page List of Illustrations iii Introduction 1 Image Processing 2 Mosaics 3 Airbrush Enhancement 12 Conclusions 31 Acknowledgemen ts 31 Bibliography . 33 ILLUSTRATIONS Page Figure 1. A "raw" picture received from Mariner 9 4 2. "Shading corrected" picture received 5 from Mariner 9 3. High-pass filtered pictur e 6 4. "Reduced Data Record" (RDR) picture 7 5. Map projection processing 8 6a . The actual shape that should be assumed by a single picture element in a hypothe- tical transformation 9 6b. The picture element of 6a represented by four picture elements in the transforma- tion array . 9 6c. The picture element of 6a represented by many picture elements in the transforma- tion array 9 7. "Shading cor rected" version of a Mariner 9 picture 10 8. "Sun-angle corrected" version of the picture in fig. 7 11 9a. ''Variable-scale" mosaic of the Tharsis (MC 9) quadr angle of Mars 13 9b. ''Variable-scale" mosaic of the Syrtis Major (MC 13) quadrangle of Mars 14 iii ILLUSTRATIONS (Cont.) Page Figure l0a. Uncontrolled mosaic of the Tharsis quadrangle of Mars 15 l0b. -
A QUALITY CONSTRAINED THEMIS DAYTIME INFRARED GLOBAL MOSAIC Jonathon R
A QUALITY CONSTRAINED THEMIS DAYTIME INFRARED GLOBAL MOSAIC Jonathon R. Hill1 and Philip R. Christensen1 1School of Earth and Space Exploration, Arizona State University, Tempe, AZ ([email protected]) Abstract #2964 OVERVIEW RESULTS OF MOSAIC IMPROVEMENTS The THEMIS daytime infrared global mosaic compiled by Hill et al. (2014) This work has resulted in the development of an [1] has been re-evaluated on an image-by-image basis in order to replace updated and quality constrained version of the THEMIS poor quality images with the highest quality images available. The resulting Day IR Global Mosaic, which has been designated quality constrained mosaic is complete from 90°N - 87.3°S and is the version 13.0. This is highest resolution and highest highest quality, highest resolution global map of Mars currently available. quality global map of Mars currently available. As an example of the improvements made throughout the global mosaic, particularly the increased emphasis on regional contrast over local contrast, the improved Syrtis BACKGROUND Major quadrangle mosaic is presented in Figure 1. The improved THEMIS Day IR Global Mosaic has also allowed updated versions of two combination global The 2001 Mars Odyssey spacecraft entered orbit around Mars on October mosaic products to be produced. An updated version of 24th, 2001 and is the longest-operating spacecraft in the history of Mars the “THEMIS Day IR with MOLA Color” global map, exploration. The Thermal Emission Imaging System (THEMIS) has been designated version 2.0, was produced by overlaying acquiring observations in both infrared and visible wavelengths since the colorized surface elevation data from the Mars Orbiter beginning of science operations in February 2002. -
CHRONOLOGY of SYRTIS MAJOR QUADRANGLE. Adele Fuller Williams, (Dept
Lunar and Planetary Science XXXVI (2005) 1441.pdf CHRONOLOGY OF SYRTIS MAJOR QUADRANGLE. Adele Fuller Williams, (Dept. of Geology and Geological Engineering, University of Idaho, Moscow, ID 83844). Introduction: A chronology of the Syrtis Major but it did not force the bend to occur. It probably only Quadrangle on Mars has been devised using geologic masked a more gradual bend or, more probably, [1], topographic, gravimetric [2], and magnetic data [4] contributed to the final outline of the boundary. along with recent narrow-angle images from the Mars The Nili Fossae are concentric to the Isidis Basin Orbiter Camera [4]. rim and are essentially contemporaneous with the A study of the Syrtis Major Quadrangle has found formation of Isidis or very slightly younger if the narrow-angle images among the more than 900 fossae formed in reaction to the initial impact. They available between 1999 and 2004 that demonstrate occur within Arabia Terra-type basement material (Fig. superposition along the boundaries between the major 2, next page). structures. Recent research of the area by Ivanov and Syrtis Major volcanics superpose the main trough Head (2003) [5] and Hiesinger and Head (2004) [6] of the Nili Fossae at its southern extremity. Lobes of has been compared to and incorporated with this study Syrtis Major volcanics also superpose Arabia Terra to [7]. the west and Isidis to the east and probably contributed Results: : The dichotomy boundary and Arabia Terra to the masking of the dichotomy boundary. The are two of the five major structural features within the inception of Syrtis Major volcanics may have preceded quadrangle (Fig. -
Hydrothermal Activity Recorded in Post Noachian-Aged Impact Craters on Mars
PUBLICATIONS Journal of Geophysical Research: Planets RESEARCH ARTICLE Hydrothermal activity recorded in post Noachian-aged 10.1002/2015JE004989 impact craters on Mars Key Points: Stuart M. R. Turner1, John C. Bridges1, Stephen Grebby2, and Bethany L. Ehlmann3 • Mars craters in post Noachian terrains studied with CRISM to determine 1Space Research Centre, Department of Physics and Astronomy, University of Leicester, Leicester, UK, 2British Geological presence of hydrated minerals 3 • Only 3 of 158 craters show Survey, Nottingham, UK, Division of Geological and Planetary Sciences and Jet Propulsion Laboratory, California Institute clay-bearing assemblages of Technology, Pasadena, California, USA • Impact hydrothermalism possible in two craters Abstract Hydrothermal systems have previously been reported in ancient Noachian and Hesperian-aged Supporting Information: craters on Mars using CRISM but not in Amazonian-aged impact craters. However, the nakhlite meteorites do • Supporting Information S1 provide evidence of Amazonian hydrothermal activity. This study uses CRISM data of 144 impact craters of • Table S1 ≥7 km diameter and 14 smaller craters (3–7 km diameter) within terrain mapped as Amazonian to search for minerals that may have formed as a result of impact-induced hydrothermal alteration or show excavation of Correspondence to: – J. C. Bridges, ancient altered crust. No evidence indicating the presence of hydrated minerals was found in the 3 7km [email protected] impact craters. Hydrated minerals were identified in three complex impact craters, located at 52.42°N, 39.86°E in the Ismenius Lacus quadrangle, at 8.93°N, 141.28°E in Elysium, and within the previously studied Stokes crater. Citation: These three craters have diameters 20 km, 62 km, and 51 km.