Energy's Place in Economic Theory
Total Page:16
File Type:pdf, Size:1020Kb
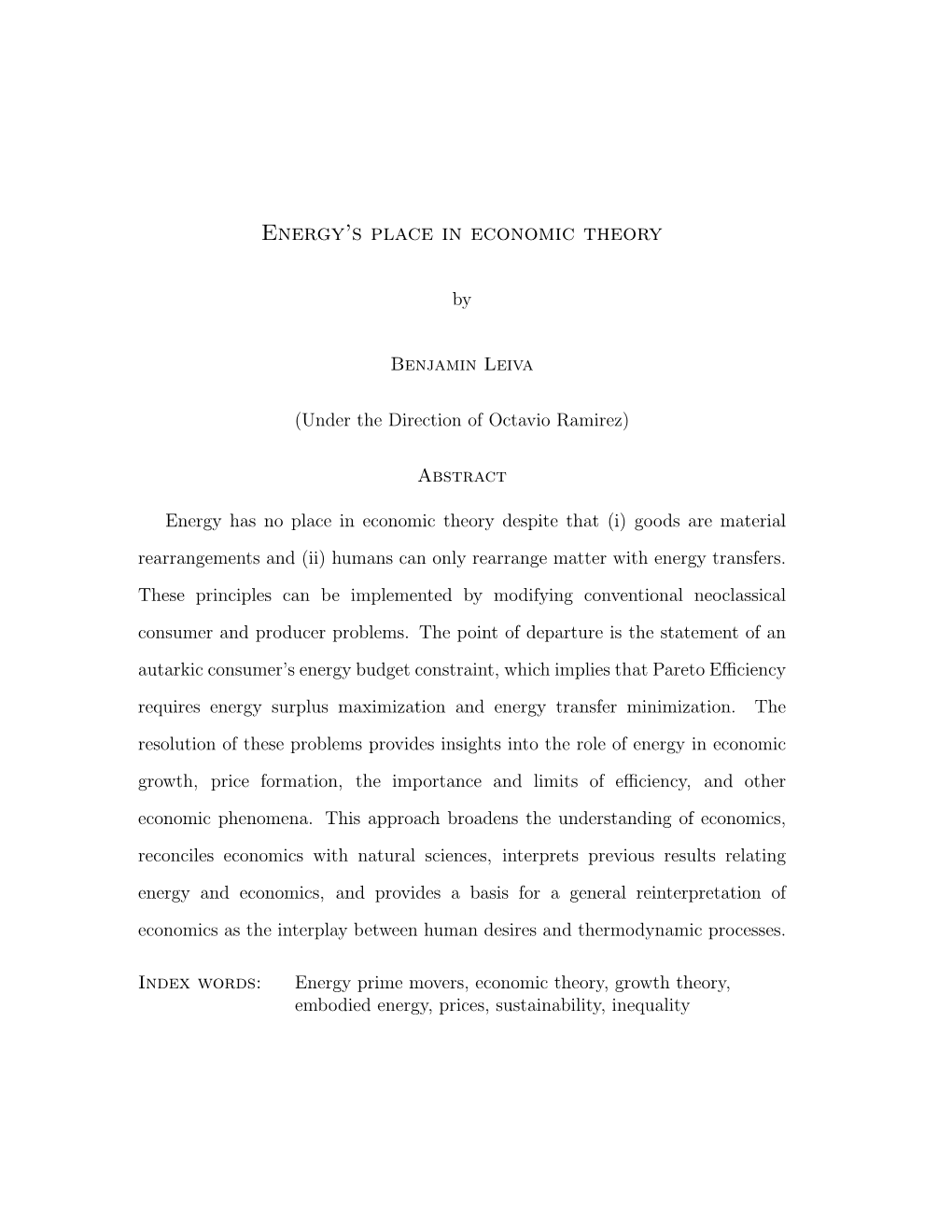
Load more
Recommended publications
-
The Austrian School in Bulgaria: a History✩ Nikolay Nenovsky A,*, Pencho Penchev B
Russian Journal of Economics 4 (2018) 44–64 DOI 10.3897/j.ruje.4.26005 Publication date: 23 April 2018 www.rujec.org The Austrian school in Bulgaria: A history✩ Nikolay Nenovsky a,*, Pencho Penchev b a University of Picardie Jules Verne, Amiens, France b University of National and World Economy, Sofia, Bulgaria Abstract The main goal of this study is to highlight the acceptance, dissemination, interpretation, criticism and make some attempts at contributing to Austrian economics made in Bulgaria during the last 120 years. We consider some of the main characteristics of the Austrian school, such as subjectivism and marginalism, as basic components of the economic thought in Bulgaria and as incentives for the development of some original theoreti- cal contributions. Even during the first few years of Communist regime (1944–1989), with its Marxist monopoly over intellectual life, the Austrian school had some impact on the economic thought in the country. Subsequent to the collapse of Communism, there was a sort of a Renaissance and rediscovery of this school. Another contribution of our study is that it illustrates the adaptability and spontaneous evolution of ideas in a different and sometimes hostile environment. Keywords: history of economic thought, dissemination of economic ideas, Austrian school, Bulgaria. JEL classification: B00, B13, B30, B41. 1. Introduction The emergence and development of specialized economic thought amongst the Bulgarian intellectuals was a process that occurred significantly slowly in comparison to Western and Central Europe. It also had its specific fea- tures. The first of these was that almost until the outset of the 20th century, the economic theories and different concepts related to them were not well known. -
How Doctrinal Are the Doctrines of Economic Thought? a Critical Evaluation
Archives of Business Research – Vol.6, No.7 Publication Date: July. 25, 2018 DOI: 10.14738/abr.67.2236. Eriemo, N. O. (2018). How Doctrinal Are The Doctrines Of Economic Thought? A Critical Evaluation. Archives of Business Research, 6(7), 34-44. How Doctrinal Are The Doctrines Of Economic Thought? A Critical Evaluation Nathanael Oke. Eriemo, PhD Department of Economics, Delta State University, Abraka, Nigeria ABSTRACT This paper is a concerted attempt to elucidate the interplay between the use of history, doctrine and theory among economists and allied managers of economic resources over ages and to evaluate the doctrinal claims in economic thought theorizing as particularly reflected in the economies of the Less Developed Countries. Thus taking economic thought as dogma, institutional and society variability in terms of desires make sweeping generalization about doctrine and persistent followership controversial and intolerable. Keywords: doctrinal, economic theory, economic doctrine, controversies INTRODUCTION Economic researchers have severally used economic theory and economic doctrine interchangeably yet they not necessarily mean the same. Economic theories refer to universally tested behaviour with respect to wealth acquisition and utilisation while economic doctrine is a principle or body of principles presented for acceptance or belief as by religious, political, scientific group; dogma. Wikipedia defined doctrine as a codification of beliefs or a body of teachings or instructions, taught principles or positions, as the essence of teaching in a given branch of knowledge or in a belief system. Arising from the foregoing, one is apt to take a position that economic thought provides the basis for economic theory formulation by man since the beginning of age. -
The Physiocrats Six Lectures on the French Économistes of the 18Th Century
The Physiocrats Six Lectures on the French Économistes of the 18th Century Henry Higgs Batoche Books Kitchener 2001 First Edition: The Macmillan Company, 1897 This Edition: Batoche Books Limited 52 Eby Street South Kitchener, Ontario N2G 3L1 Canada email: [email protected] ISBN: 1-55273-064-6 Contents Preface ............................................................................................... 5 I: Rise of the School. .......................................................................... 6 II: The School and Its Doctrines. ..................................................... 17 III: The School and Its Doctrines (contd.) ....................................... 29 IV: Activities of the School. ............................................................. 43 V: Opponents of the School. ............................................................ 55 VI: Influence of the School. ............................................................. 66 Appendix .......................................................................................... 77 Authorities ....................................................................................... 80 Notes ................................................................................................ 82 Preface This little volume consists of lectures delivered before the London School of Economics in May and June of the present year. Impossible though it was found to give a truly adequate account of the Physiocrats in these six lectures, it has been thought that they may perhaps furnish -
HISTORY of ECONOMIC THOUGHT a Selected Bibliography John F
HISTORY OF ECONOMIC THOUGHT A Selected Bibliography John F. Henry Department of Economics California State University, Sacramento A. Texts and Commentaries of a General Nature (The following list is not meant to be exhaustive, but does represent general accounts from varying points of view. The student should also examine the bibliographies in these works, particularly that in Spiegel. There are also a number of series studies now available, notably from Edward Elgar publisher: Perspectives in the History of Economic Thought which features papers presented at the annual History of Economics Society meeting (9 vols); Pioneers in Economics, Marc Blaug, ed., which contains papers on particular economists (currently at 46 titles); Schools of Thought in Economics, Marc Blaug, general ed. which contains (currently 11) volumes of essays on particular general approaches in economics. Routledge is publishing a Critical Assessments series, edited by John Wood featuring articles written on specific economists: currently, volumes on Joan Robinson, Leontief, Say, and Pareto are published. Routledge also publishes a “Library of 20th Century Economists,” organized around themes–The Chicago Tradition, Socialism and the Market, Origins of Macroeconomics, etc. And, the firm has a “Critical Reviews” and Critical Responses” series. Macmillan has a new series on Contemporary Economists, edited by John Pheby. Finally, Pickering and Chatto (UK) is publishing series on various topics that collect articles written over a several hundred year period: Hageman, H., ed., Business Cycle Theory (4 vols); Emmett, R., Reactions to the South Sea Bubble, the Mississippi Scheme, and the Tulip Mania Affair (3 vols); White, L, ed., The History of Gold and Silver (3vols); O’Brien, D., The History of Taxation (8 vols); Bridel, P., The Foundations of Price Theory (6 vols); Barber, W., et al., eds, Early American Thought (6 vols); Samuels, W., ed., Law and Economics (2 vols); Capie, F., ed., History of Banking, 1650-1850 (10 vols); Ross, D., History of Banking II, 1844-1959 (10 vols). -
PROFESSIONALIZING ECONOMICS: the 'Marginalist Revolution' in Historical Context Michael A. Bernstein Department of History 0
PROFESSIONALIZING ECONOMICS: The ‘Marginalist Revolution’ in Historical Context Michael A. Bernstein Department of History 0104 University of California, San Diego 9500 Gilman Drive La Jolla, California 92093-0104 [USA] [Phone: 858-534-1070] [Fax: 858-534-7283] [[email protected]] 2 Economic analysis, serving for two centuries to win an understanding of the Nature and Causes of the Wealth of Nations, has been fobbed off with another bride -- a Theory of Value. There were no doubt deep-seated political reasons for the substitution but there was also a purely technical, intellectual reason. -- Joan Robinson [1956]i If the last years of the nineteenth century witnessed the first, genuine articulation of a professional self-consciousness among American economists, then they also demarcated the establishment of an altogether novel protocol for those experts. This new agenda, developed with increasing rigor and authority as the twentieth century beckoned, began a significant reorientation of the field's object of study while at the same time it reconfigured long-standing perceptions of the history of economic thought as a whole. Scientific sophistication necessarily involved a revision of practice, yet it also encouraged the articulation of new perceptions of its pedigree.ii Linking the object of study with particular and venerable authorities from the ages was of singular importance to the successful construction of a distinctly professional knowledge. Framing that understanding in a particular way was the result of both a social and an intellectual process. With their most apparent and seemingly immediate intellectual roots in the moral philosophy of the eighteenth and nineteenth centuries, modern economists were (and are) eager to invoke validation by impressive forebears and traditions. -
History of Economic Thought Professor Lynne Kiesling Tuesday-Thursday 4:30-5:50 Krannert G012
Economics 455 Fall 2018 History of Economic Thought Professor Lynne Kiesling Tuesday-Thursday 4:30-5:50 Krannert G012 Office location: 345 Krannert Hall Email: [email protected] Office hours: Thursday 2:00-4:00 By appointment Readings: Jürg Niehans, A History of Economic Theory (HET) – required Adam Smith, The Wealth of Nations, Liberty Press edition (WN) – required Bucholz, New Ideas from Dead Economists – required All other readings available on the course Blackboard website Readings marked with an asterisk (*) are required and are all included in the assigned texts or the online readings. The other references are optional additional reading, all of which should also be available in the library if you want to explore something in more depth. NOTE: You should also ensure that you have access to intermediate micro and macro texts, as you may feel the need to brush up on the theory that we will be discussing. For example, I will take as given that you are familiar with the IS/LM framework of the Keynesian model, and the contrasting classical model, when we discuss Keynes. McCloskey’s Applied Theory of Price is an intermediate micro book that’s available online if you don’t still have yours from 340: http://www.deirdremccloskey.com/docs/price.pdf Description: The development of economic thought and economic methodology from the advent of the mercantilists to the formation of current schools of economics in the early 20th century. The course will focus on economics as an evolving body of thought and an ongoing conversation, with strong emphasis the movement from classical economics to neoclassical economics in the 19th century as a foundation for modern economic theory. -
Foucault on Neoliberalism and the Art of Governing
FOUCAULT ON NEOLIBERALISM AND THE ART OF GOVERNING John Protevi Department of French Studies Louisiana State University Final draft: 28 June 2009. Comments welcome at [email protected] Extract from "What does Foucault Think is New about Neo-Liberalism?" Published in Pli: Warwick Journal of Philosophy, vol. 21 http://www.protevi.com/john/Foucault_28June2009.pdf In conducting his genealogy of governmentality as a mode of social power, Foucault begins with an analysis of "pastoral power" in Christian history as a concern with both the individual and the whole. After distinguishing the Christian pastorate from the theme of the shepherd of men in Hebrew and Greek thought, Foucault dwells on the famous paradoxes of the good shepherd: he must care for the whole flock, but he must also leave the whole flock to tend to the lost sheep, whose individual salvation is his task. Foucault thus established pastoral power as one of the historically first individualizing practices, the grid by which he had previously analyzed the human sciences, which come into being with 19th century disciplinary society (STP 132F / 128E). We should recall that the move to governmentality is a move "outside" the state. In this way, Foucault can show the great turning point (péripétie) that is the "statification" (étatisation) of governmentality (STP 253F / 248E). The first great episode here is the administrative / absolute state and its political rationality of raison d'Etat, analyzed in Sécurité, territoire, population. This is only a nascent form of the political art of governing men, as it is still caught in the paradigm of sovereignty (STP 105F / 102E). -
John Stuart Mill and China: Peeking Behind China’S Stationary State
John Stuart Mill and China: Peeking Behind China’s Stationary State Yue Xiao* Abstract Current literature on John Stuart Mill’s writings about Asia has mainly focused on his influence in India because of Mill’s 35-year career in the East India Company. Scholars in both China and the West have not paid attention to Mill’s views on China. This paper delves into Mill’s notion of China’s stationary state and categorizes Mill’s discussion of China into three major topics: (1) capital accumulation, (2) liberty and individuality, and (3) democratic government. Mill made an empirical analysis of the relationship between China’s high interest rate and the desire for capital accumulation. He went on to explore the negative connection between China’s “despotism of custom” and individual liberty. Finally, he considered the autocratic government and the lack of civil rights. Mill traced China’s stationary state from the time preferences of people to broad institutional failures. Keywords: John Stuart Mill, China, stationary state JEL Codes: B1, B3 * Correspondence may be addressed to Yue Xiao ([email protected]), a postdoctoral researcher at the School of Business Administration at Zhongnan University of Economics and Law, and a research scholar at the Department of Economics at the University of Illinois at Chicago (UIC). I would like to thank the History of Economics Society for awarding this paper as the Best Conference Paper by a Young Scholar in 2019. I thank Joseph Persky at UIC for his inspiration to work on this topic, as well as his generous support over the years. -
Physiocracy: a Viewpoint of the Role of Agricultural Production in a Macroeconomic System
University of Montana ScholarWorks at University of Montana Graduate Student Theses, Dissertations, & Professional Papers Graduate School 1994 Physiocracy: A viewpoint of the role of agricultural production in a macroeconomic system Ronald A. de Yong The University of Montana Follow this and additional works at: https://scholarworks.umt.edu/etd Let us know how access to this document benefits ou.y Recommended Citation de Yong, Ronald A., "Physiocracy: A viewpoint of the role of agricultural production in a macroeconomic system" (1994). Graduate Student Theses, Dissertations, & Professional Papers. 8674. https://scholarworks.umt.edu/etd/8674 This Thesis is brought to you for free and open access by the Graduate School at ScholarWorks at University of Montana. It has been accepted for inclusion in Graduate Student Theses, Dissertations, & Professional Papers by an authorized administrator of ScholarWorks at University of Montana. For more information, please contact [email protected]. m Maureen and Mike MANSFIELD LIBRARY TheMontana University of Permission is granted by the author to reproduce this material in its entirety, provided that this material is used for scholarly purposes and is properly cited in published works and reports. Please check ”Yes ” or **No and provide signature Yes, I grant permission No, I do not grant permission Author’s Signature :or commercial purposes or financial gain may be undeitaken author’s explicit consent.____________________________ Reproduced with permission of the copyright owner. Further reproduction prohibited without permission. Reproduced with permission of the copyright owner. Further reproduction prohibited without permission. Physiocracy : A Viewpoint of the Role of Agricultural Production in a Macroeconomic System by Ronald A. -
Austrian Economics As Political Philosophy J. Mikael Olsson
S TOCKHOLM STUDIES IN POLITICS 161 Austrian Economics as Political Philosophy J. Mikael Olsson Austrian Economics as Political Philosophy J. Mikael Olsson ©J. Mikael Olsson, Stockholm University 2015 ISSN 0346-6620 ISBN 978-91-7649-062-4 Printed in Sweden by US-AB, Stockholm 2015 Distributor: Department of Political Science, Stockholm University. Cover image: Micaela Adolfsson (based on “Under the Thumb” by Thomas Nast, 1871). Contents I. Introduction ............................................................................................... 7 Aims of the Study ...................................................................................... 12 Some Words on Method ............................................................................ 17 The Metaethical Dimension ...................................................................... 22 Earlier Research ........................................................................................ 31 Sources ...................................................................................................... 33 Disposition ................................................................................................ 34 Part 1: Economics ........................................................................................ 35 II. A Brief History of Economic Thought ................................................. 37 Mercantilism and Its Critics ...................................................................... 38 Quesnay and Smith ................................................................................... -
Topologies of Power: Foucault's Analysis of Political Government Beyond 'Governmentality' Stephen J
Theory, Culture & Society http://tcs.sagepub.com/ Topologies of Power: Foucault's Analysis of Political Government beyond 'Governmentality' Stephen J. Collier Theory Culture Society 2009 26: 78 DOI: 10.1177/0263276409347694 The online version of this article can be found at: http://tcs.sagepub.com/content/26/6/78 Published by: http://www.sagepublications.com On behalf of: The TCS Centre, Nottingham Trent University Additional services and information for Theory, Culture & Society can be found at: Email Alerts: http://tcs.sagepub.com/cgi/alerts Subscriptions: http://tcs.sagepub.com/subscriptions Reprints: http://www.sagepub.com/journalsReprints.nav Permissions: http://www.sagepub.com/journalsPermissions.nav Citations: http://tcs.sagepub.com/content/26/6/78.refs.html >> Version of Record - Dec 9, 2009 What is This? Downloaded from tcs.sagepub.com at Bobst Library, New York University on June 12, 2014 078-108 TCS347694 Collier_Article 156 x 234mm 16/11/2009 10:04 Page 78 Topologies of Power Foucault’s Analysis of Political Government beyond ‘Governmentality’ Stephen J. Collier Abstract The publication of Michel Foucault’s lectures at the Collège de France in the late 1970s has provided new insight into crucial developments in his late work, including the return to an analysis of the state and the introduction of biopolitics as a central theme. According to one dominant interpretation, these shifts did not entail a fundamental methodological break; the approach Foucault developed in his work on knowledge/power was simply applied to new objects. The present article argues that this reading – which is colored by the overwhelming privilege afforded to Discipline and Punish in secondary literature – obscures an important modification in Foucault’s method and diagnostic style that occurred between the introduction of biopolitics in 1976 (in Society Must Be Defended) and the lectures of 1978 (Security, Territory, Population) and 1979 (Birth of Biopolitics). -
Mr Marx and the Neoclassics
Munich Personal RePEc Archive Mr Marx and the neoclassics Freeman, Alan the University of Greenwich July 1996 Online at https://mpra.ub.uni-muenchen.de/1291/ MPRA Paper No. 1291, posted 04 Feb 2007 UTC MR MARX AND THE NEOCLASSICS Alan Freeman University of Greenwich September 1996 ABSTRACT This article, presented to the Annual Conference of the History of Economics Society, Vancouver July 1996, gives a historical analysis of the origins of the general equilibrium or comparative static approach and demonstrates that economic thought as a whole is divided, in each of its schools of thought, between the equilibrium paradigm and its alternative, the temporal paradigm. This applies across the board with, for example, the divergence between Post-Keynesian or Kaleckian economics, between Austrian economics and Walrasian general equilibrium, and in many other contexts . The article demonstrates the difference between the equilibrium and temporal approach using a demonstration of ‘adjustment’ effects in a simple corn-cycle model. It goes on to analyse the reasons why, in the history of thought, adjustment or dynamic effects have been considered as ignorable when in fact they are not. It suggests that the traditional division of the succession of ideas in economic thought – physiocracy, the classics, Marx, marginalism – needs to be reviewed in this light, and argues for a reconsideration of the contribution of Marx to economics, placing him as the first and in many ways the most consistent in a suppressed non-equilibrium tradition in economic thought. It suggests that in this light, Marx has more in common with Austrian and Post-Keynesian thinking than with Ricardo and Smith, among whose ranks he is normally and commonly grouped.