The F-Box Protein MAX2 Contributes to Resistance to Bacterial
Total Page:16
File Type:pdf, Size:1020Kb
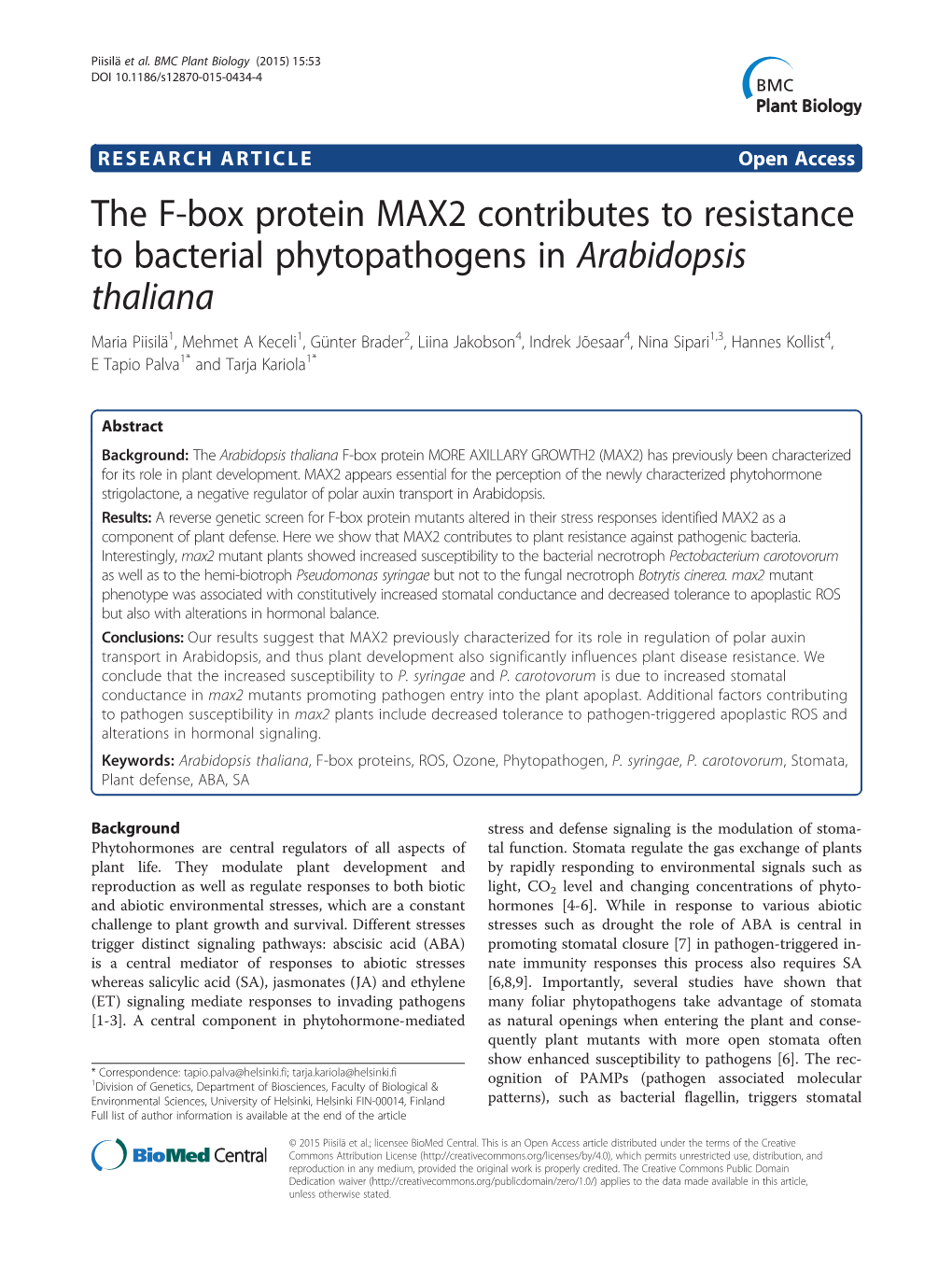
Load more
Recommended publications
-
Identification of Multiple Salicylic Acid-Binding Proteins Using Two High Throughput Screens
UC Davis UC Davis Previously Published Works Title Identification of multiple salicylic acid-binding proteins using two high throughput screens. Permalink https://escholarship.org/uc/item/3kb0g6gm Journal Frontiers in plant science, 5(JAN) ISSN 1664-462X Authors Manohar, Murli Tian, Miaoying Moreau, Magali et al. Publication Date 2014 DOI 10.3389/fpls.2014.00777 Peer reviewed eScholarship.org Powered by the California Digital Library University of California ORIGINAL RESEARCH ARTICLE published: 12 January 2015 doi: 10.3389/fpls.2014.00777 Identification of multiple salicylic acid-binding proteins using two high throughput screens Murli Manohar 1‡, Miaoying Tian 1† ‡, Magali Moreau 1† ‡, Sang-Wook Park 1†, Hyong Woo Choi 1, Zhangjun Fei 1,2, Giulia Friso 3,MuhammedAsif1†, Patricia Manosalva 1, Caroline C. von Dahl 1†, Kai Shi 1†, Shisong Ma 4, Savithramma P.Dinesh-Kumar 4, Inish O’Doherty 1†, Frank C. Schroeder 1, Klass J. van Wijk 3 and Daniel F. Klessig 1* 1 Boyce Thompson Institute for Plant Research, Cornell University, Ithaca, NY, USA 2 Plant, Soil, and Nutrition Laboratory, United States Department of Agriculture, Ithaca, NY, USA 3 Department of Plant Biology, Cornell University, Ithaca, NY, USA 4 Department of Plant Biology and Genome Center, University of California, Davis, Davis, CA, USA Edited by: Salicylic acid (SA) is an important hormone involved in many diverse plant processes, Loreto Holuigue, Pontificia including floral induction, stomatal closure, seed germination, adventitious root initiation, Universidad Católica de Chile, Chile and thermogenesis. It also plays critical functions during responses to abiotic and biotic Reviewed by: stresses. The role(s) of SA in signaling disease resistance is by far the best studied Steven H. -
The Complex Origins of Strigolactone Signalling in Land Plants
bioRxiv preprint doi: https://doi.org/10.1101/102715; this version posted January 25, 2017. The copyright holder for this preprint (which was not certified by peer review) is the author/funder, who has granted bioRxiv a license to display the preprint in perpetuity. It is made available under aCC-BY-NC-ND 4.0 International license. Article - Discoveries The complex origins of strigolactone signalling in land plants Rohan Bythell-Douglas1, Carl J. Rothfels2, Dennis W.D. Stevenson3, Sean W. Graham4, Gane Ka-Shu Wong5,6,7, David C. Nelson8, Tom Bennett9* 1Section of Structural Biology, Department of Medicine, Imperial College London, London, SW7 2Integrative Biology, 3040 Valley Life Sciences Building, Berkeley CA 94720-3140 3Molecular Systematics, The New York Botanical Garden, Bronx, NY. 4Department of Botany, 6270 University Boulevard, Vancouver, British Colombia, Canada 5Department of Medicine, University of Alberta, Edmonton, Alberta, Canada 6Department of Biological Sciences, University of Alberta, Edmonton, Alberta, Canada 7BGI-Shenzhen, Beishan Industrial Zone, Yantian District, Shenzhen, China. 8Department of Botany and Plant Sciences, University of California, Riverside, CA 92521 USA 9School of Biology, University of Leeds, Leeds, LS2 9JT, UK *corresponding author: Tom Bennett, [email protected] Running title: Evolution of strigolactone signalling 1 bioRxiv preprint doi: https://doi.org/10.1101/102715; this version posted January 25, 2017. The copyright holder for this preprint (which was not certified by peer review) is the author/funder, who has granted bioRxiv a license to display the preprint in perpetuity. It is made available under aCC-BY-NC-ND 4.0 International license. ABSTRACT Strigolactones (SLs) are a class of plant hormones that control many aspects of plant growth. -
The Flowering Hormone Florigen Accelerates Secondary Cell Wall
bioRxiv preprint doi: https://doi.org/10.1101/476028; this version posted November 27, 2018. The copyright holder for this preprint (which was not certified by peer review) is the author/funder. All rights reserved. No reuse allowed without permission. 1 The Flowering Hormone Florigen Accelerates Secondary Cell Wall Biogenesis to Harmonize Vascular Maturation with Reproductive Development Akiva Shalit-Kaneh*1, Tamar Eviatar–Ribak*1, Guy Horev*2, Naomi Suss1, Roni Aloni3, Yuval Eshed4, Eliezer Lifschitz 1,5 1Department of Biology, Technion IIT 2Lorey I. Lokey Center for Life Sciences & Engineering, Technion 3 School of Plant Sciences and Food Security, Tel Aviv University, Tel Aviv 69978, Israel 4Department of Plant and Environmental Sciences Weizmann Institute of Science, Rehovot, Israel *Equal contribution 5 Corresponding author [email protected] Developmental Highlights - Florigen accelerates SCWB: A prime case for a long-range regulation of a complete metabolic network by a plant hormone. - The dual acceleration of flowering and vascular maturation by Florigen provides a paradigm for a dynamic regulation of global, independent, developmental programs. - The growth termination functions of florigen and the auto-regulatory mechanism for its production and distribution provide a communication network enveloping the shoot system. - A stable florigen provides a possible mechanism for the quantitative regulation of flowering - Lateral stimulation of xylem differentiation links the phloem-travelling florigen with the annual rings in trunks. - MADS genes are common relay partners in Florigen circuits; vascular maturation in stems and reproductive transition in apical meristems. bioRxiv preprint doi: https://doi.org/10.1101/476028; this version posted November 27, 2018. The copyright holder for this preprint (which was not certified by peer review) is the author/funder. -
Multiple Pathways Regulate Shoot Branching Catherine Rameau, Jessica Bertheloot, Nathalie Leduc, Bruno Andrieu, Fabrice Foucher, Soulaiman Sakr
Multiple pathways regulate shoot branching Catherine Rameau, Jessica Bertheloot, Nathalie Leduc, Bruno Andrieu, Fabrice Foucher, Soulaiman Sakr To cite this version: Catherine Rameau, Jessica Bertheloot, Nathalie Leduc, Bruno Andrieu, Fabrice Foucher, et al.. Mul- tiple pathways regulate shoot branching. Frontiers in Plant Science, Frontiers, 2015, 5, pp.741. 10.3389/fpls.2014.00741. hal-01168759 HAL Id: hal-01168759 https://hal.archives-ouvertes.fr/hal-01168759 Submitted on 28 May 2020 HAL is a multi-disciplinary open access L’archive ouverte pluridisciplinaire HAL, est archive for the deposit and dissemination of sci- destinée au dépôt et à la diffusion de documents entific research documents, whether they are pub- scientifiques de niveau recherche, publiés ou non, lished or not. The documents may come from émanant des établissements d’enseignement et de teaching and research institutions in France or recherche français ou étrangers, des laboratoires abroad, or from public or private research centers. publics ou privés. REVIEW ARTICLE published: 13 January 2015 doi: 10.3389/fpls.2014.00741 Multiple pathways regulate shoot branching Catherine Rameau 1,2*, Jessica Bertheloot 3, Nathalie Leduc 4, Bruno Andrieu 5,6, Fabrice Foucher 3 and Soulaiman Sakr 7 1 Institut Jean-Pierre Bourgin, INRA, UMR 1318, ERL CNRS 3559, Saclay Plant Sciences, Versailles, France 2 Institut Jean-Pierre Bourgin, AgroParisTech, UMR 1318, ERL CNRS 3559, Saclay Plant Sciences, Versailles, France 3 UMR1345 IRHS, INRA, SFR 4207 QUASAV, Beaucouzé, France 4 UMR1345 IRHS, Université d’Angers, SFR 4207 QUASAV, Angers, France 5 UMR1091 EGC, INRA, Thiverval-Grignon, France 6 UMR1091 EGC, AgroParisTech, Thiverval-Grignon, France 7 UMR1345 IRHS, Agrocampus-Ouest, SFR 4207 QUASAV, Angers, France Edited by: Shoot branching patterns result from the spatio-temporal regulation of axillary bud Alexandra Jullien, AgroParisTech, outgrowth. -
Three Mutations Repurpose a Plant Karrikin
Three mutations repurpose a plant karrikin receptor to a strigolactone receptor Amir Arellano-Saab, Shigeo Toh, Hasan Al Galib, Wenda Zhao, Stefan Schuetz, James Michael Bradley, Asrinus Subha, Zhenhua Xu, Alexandre de Saint Germain, Claresta Adityani, et al. To cite this version: Amir Arellano-Saab, Shigeo Toh, Hasan Al Galib, Wenda Zhao, Stefan Schuetz, et al.. Three mu- tations repurpose a plant karrikin receptor to a strigolactone receptor. Proceedings of the National Academy of Sciences of the United States of America , National Academy of Sciences, 2021, 118 (30), pp.e2103175118. 10.1073/pnas.2103175118. hal-03299064 HAL Id: hal-03299064 https://hal.archives-ouvertes.fr/hal-03299064 Submitted on 26 Jul 2021 HAL is a multi-disciplinary open access L’archive ouverte pluridisciplinaire HAL, est archive for the deposit and dissemination of sci- destinée au dépôt et à la diffusion de documents entific research documents, whether they are pub- scientifiques de niveau recherche, publiés ou non, lished or not. The documents may come from émanant des établissements d’enseignement et de teaching and research institutions in France or recherche français ou étrangers, des laboratoires abroad, or from public or private research centers. publics ou privés. 1 Three mutations repurpose a plant karrikin receptor to a strigolactone receptor 2 3 Amir Arellano-Saab1,7, Shigeo Toh2,7, Hasan Galib1, Wenda Zhao1, Stefan Schuetz1, James 4 Michael Bradley1, Asrinus Subha1, Alexandre de Saint Germain3, Claresta Adityani1, Michael 5 Bunsick1, Hayley McKay1, François-Didier Boyer4, Christopher S. P. McErlean5, Peter 6 McCourt1,8 Peter J. Stogios6,8 and Shelley Lumba1,8 7 8 1 Department of Cell & Systems Biology, University of Toronto, 25 Willcocks Street, Toronto 9 M5S 3B2, Canada 10 11 2 Department of Environmental Bioscience, School of Agriculture, Meijo University 12 1-501 Shiogamaguchi, Tenpaku-ku, Nagoya, Japan, 468-8502 13 14 3 Institut Jean-Pierre Bourgin, INRAE, AgroParisTech, Université Paris-Saclay, 78000, 15 Versailles, France. -
Quantification of Karrikins in Smoke Water Using Ultra-High Performance Liquid Chromatography-Tandem Mass Spectrometry
PALACKÝ UNIVERSITY IN OLOMOUC Faculty of Science Laboratory of Growth Regulators & Department of Chemical Biology New methods for karrikin analysis Ph.D. thesis Author : Mgr. Jakub Hrdlička Study programme: P1527 / Biology Study branch: 1501V019 / Experimental Biology Supervisor: Mgr. Karel Doležal, Dr., DSc. Consultant: Doc. Mgr. Ondřej Novák, Ph.D. Bibliographical identification Author’s first name and surname Mgr. Jakub Hrdlička Title of thesis New methods for karrikin analysis Type of thesis Ph.D. Department Laboratory of Growth Regulators Supervisor Mgr. Karel Doležal, Dr., DSc. The year of presentation 2021 Abstract Karrikins (KARs), chemically butenolide derivatives, are plant growth regulators that promote seed germination and the subsequent growth and development of seedlings of many plant species in extremely low concentration. This thesis presents a method development of a new analytical approach for quantification of KARs using ultra-high performance liquid chromatography with tandem mass spectrometry. Due to the employment of liquid chromatography-mass spectrometry using reverse phase-based separation and quantification by multiple reaction monitoring we developed, validated and applied a fast, specific and sensitive method which will give us the possibility to study these new interesting class of biostimulants in more detail. Keywords Karrikins, UHPLC–ESI-MS/MS, UniSpray Number of pages 48 Number of supplements 3 Language English 2 Acknowledgement This research was performed at the Laboratory of Growth Regulators and Department of Chemical Biology by Palacký University in Olomouc under the supervision of Mgr. Karel Doležal, Dr., DSc.. His support, understanding, patience and encouragement are greatly appreciated. This work would not exist without the excellent scientific supervision and great help of doc. -
Multi-Omics Strategies for Decoding Smoke-Assisted Germination Pathways and Seed Vigour
International Journal of Molecular Sciences Review Multi-Omics Strategies for Decoding Smoke-Assisted Germination Pathways and Seed Vigour Utpal Bose 1 , Angéla Juhász 2 , James A. Broadbent 1, Setsuko Komatsu 3,* and Michelle L. Colgrave 1,2,* 1 CSIRO Agriculture and Food, 306 Carmody Rd, St Lucia, QLD 4067, Australia; [email protected] (U.B.); [email protected] (J.A.B.) 2 Australian Research Council Centre of Excellence for Innovations in Peptide and Protein Science, School of Science, Edith Cowan University, Joondalup, WA 6027, Australia; [email protected] 3 Department of Environmental and Food Sciences, Fukui University of Technology, Fukui 910-8505, Japan * Correspondence: [email protected] (S.K.); [email protected] (M.L.C.); Tel.: +61-7-3214-2697 (M.L.C.) Received: 11 September 2020; Accepted: 9 October 2020; Published: 12 October 2020 Abstract: The success of seed germination and the successful establishment of seedlings across diverse environmental conditions depends on seed vigour, which is of both economic and ecologic importance. The smoke-derived exogenous compound karrikins (KARs) and the endogenous plant hormone strigolactone (SL) are two classes of butanolide-containing molecules that follow highly similar signalling pathways to control diverse biological activities in plants. Unravelling the precise mode-of-action of these two classes of molecules in model species has been a key research objective. However, the specific and dynamic expression of biomolecules upon stimulation by these signalling molecules remains largely unknown. Genomic and post-genomic profiling approaches have enabled mining and association studies across the vast genetic diversity and phenotypic plasticity. -
Strigolactones Inhibit Auxin Feedback on PIN-Dependent Auxin Transport
ARTICLE https://doi.org/10.1038/s41467-020-17252-y OPEN Strigolactones inhibit auxin feedback on PIN-dependent auxin transport canalization ✉ Jing Zhang 1 , Ewa Mazur 2,3, Jozef Balla4,5, Michelle Gallei6, Petr Kalousek5, Zuzana Medveďová4, Yang Li1, Yaping Wang1, Tomáš Prát 6, Mina Vasileva6, Vilém Reinöhl4, Stanislav Procházka4, Rostislav Halouzka7, ✉ Petr Tarkowski7, Christian Luschnig 8, Philip B. Brewer 9 &Jiří Friml 6 Directional transport of the phytohormone auxin is a versatile, plant-specific mechanism 1234567890():,; regulating many aspects of plant development. The recently identified plant hormones, strigolactones (SLs), are implicated in many plant traits; among others, they modify the phenotypic output of PIN-FORMED (PIN) auxin transporters for fine-tuning of growth and developmental responses. Here, we show in pea and Arabidopsis that SLs target processes dependent on the canalization of auxin flow, which involves auxin feedback on PIN subcellular distribution. D14 receptor- and MAX2 F-box-mediated SL signaling inhibits the formation of auxin-conducting channels after wounding or from artificial auxin sources, during vasculature de novo formation and regeneration. At the cellular level, SLs interfere with auxin effects on PIN polar targeting, constitutive PIN trafficking as well as clathrin-mediated endocytosis. Our results identify a non-transcriptional mechanism of SL action, uncoupling auxin feedback on PIN polarity and trafficking, thereby regulating vascular tissue formation and regeneration. 1 State Key Laboratory of Plant Physiology and Biochemistry, College of Biological Sciences, China Agricultural University, Beijing 100193, China. 2 University of Silesia in Katowice, Faculty of Natural Sciences, Institute of Biology, Biotechnology and Environmental Protection, Jagiellońska 28, 40-032 Katowice, Poland. -
Strigolactone and Karrikin Signal Perception: Receptors, Enzymes, Or Both?
REVIEW ARTICLE published: 28 December 2012 doi: 10.3389/fpls.2012.00296 Strigolactone and karrikin signal perception: receptors, enzymes, or both? Bart J. Janssen* and Kimberley C. Snowden Plant Development Team, Breeding and Genomics, Plant & Food Research Institute of New Zealand, Auckland, New Zealand Edited by: The signaling molecules strigolactone (SL) and karrikin are involved in seed germination, Patricia Springer, University of development of axillary meristems, senescence of leaves, and interactions with arbuscular California at Riverside, USA mycorrhizal fungi. The signal transduction pathways for both SLs and karrikins require the Reviewed by: same F-box protein (MAX2) and closely related α/β hydrolase fold proteins (DAD2 and KAI2). Peter McCourt, University of Toronto, Canada The crystal structure of DAD2 has been solved revealing an α/β hydrolase fold protein with Thomas Greb, Gregor Mendel an internal cavity capable of accommodating SLs. DAD2 responds to the SL analog GR24 by Institute, Austria changing conformation and binding to MAX2 in a GR24 concentration-dependent manner. *Correspondence: DAD2 can also catalyze hydrolysis of GR24. Structure activity relationships of analogs Bart J. Janssen, Plant Development indicate that the butenolide ring common to both SLs and karrikins is essential for biological Team, Breeding and Genomics, Plant and Food Research Institute of New activity, but the remainder of the molecules can be significantly modified without loss of Zealand, Private Bag 92169, Auckland activity. The combination of data from the study of DAD2, KAI2, and chemical analogs of 1142, New Zealand. SLs and karrikins suggests a model for binding that requires nucleophilic attack by the active e-mail: Bart.Janssen@plantandfood. -
Positive Effects of Karrikin on Seed Germination of Three Medicinal Herbs Under Drought Stress Shiraz University M
Iran Agricultural Research (2016) 35(2) 57-64 Positive effects of karrikin on seed germination of three medicinal herbs under drought stress Shiraz University M. MousaviNik1, A. Jowkar2* , A. RahimianBoogar2 1Department of Agronomy, College of Agriculture, University of Zabol, Zabol, I. R. Iran 2 Department of Horticultural Science, College of Agriculture, Shiraz University, Shiraz, I. R. Iran *Corresponding Author: [email protected] ARTICLE INFO Article history: ABSTRACT- Improper seed germination is a serious challenge for medicinal herbs under drought stress conditions in semi-arid and arid regions. A new group of plant Received 10 March 2015 growth regulators known as karrikins have been proved useful to improve seed 2 2016 Accepted May germination in some plants. In this regard, the effects of karrikin on seed germination Available online 3 August 2016 and vigor of three medicinals under drought stress were examined. Seeds of Trachyspermum copticum (L.) Link, Foeniculum vulgare Miller, and Cuminum Keywords: cyminum L. were sowed under drought treatments (-1, -1.5, -2 and -2.5 MPa of PEG Cuminum cyminum 6000), with and without synthetic Karrikin GR24 (10µM). Results indicated increasing Foeniculum vulgare osmotic pressure seed germination characteristics were severely reduced; however, Seed vigor karrikin significantly increased the seed germination of the three herbs. The application Strigolactone of karrikin in all drought conditions significantly increased germination percentage, Trachyspermum copticum germination rate, germination index, seedling vigor, shoot length and radicle length of all herb seedlings. Ajwain (T. copticum) showed a greater drought tolerance compared to the other medicinal species. INTRODUCTION Drought stress is the major limiting factor for plant germination of many plant species (Halford, 2010; production in semi-arid and arid regions. -
F-Box Protein MAX2 Has Dual Roles in Karrikin and Strigolactone Signaling in Arabidopsis Thaliana
F-box protein MAX2 has dual roles in karrikin and strigolactone signaling in Arabidopsis thaliana David C. Nelsona, Adrian Scaffidib, Elizabeth A. Dunc, Mark T. Watersa, Gavin R. Flemattib, Kingsley W. Dixond,e, Christine A. Beveridgec, Emilio L. Ghisalbertib, and Steven M. Smitha,b,1 aAustralian Research Council Centre of Excellence in Plant Energy Biology, bSchool of Biomedical, Biomolecular and Chemical Sciences, and dSchool of Plant Biology, University of Western Australia, Crawley WA 6009, Australia; and cSchool of Biological Sciences, University of Queensland, St. Lucia QLD 4072, Australia; and eKings Park and Botanic Garden, West Perth WA 6005, Australia Edited* by Peter H. Quail, University of California at Berkeley, Albany, CA, and approved April 19, 2011 (received for review January 18, 2011) Smoke is an important abiotic cue for plant regeneration in Karrikins share partial structural similarity with strigolactones postfire landscapes. Karrikins are a class of compounds discovered (Fig. 1A), a family of phytohormones present in root exudates that in smoke that promote seed germination and influence early de- stimulate germination of parasitic weeds (Orobanche and Striga velopment of many plants by an unknown mechanism. A genetic spp.) (12, 13) and enhance hyphal branching in arbuscular mycor- screen for karrikin-insensitive mutants in Arabidopsis thaliana rhizal fungi (14). Additionally, strigolactones or a strigolactone- revealed that karrikin signaling requires the F-box protein MAX2, derived signal repress shoot branching. For the remainder of this which also mediates responses to the structurally-related strigolac- article, we will simply refer to the active signal as strigolactone. As tone family of phytohormones. Karrikins and the synthetic strigo- demonstrated by an array of mutants in pea, rice, petunia, and lactone GR24 trigger similar effects on seed germination, seedling A. -
1 Karrikin-Sensing Protein KAI2 Is a New Player in Regulating Root Growth Patterns 2 3 4 Stéphanie M
bioRxiv preprint doi: https://doi.org/10.1101/195891; this version posted September 29, 2017. The copyright holder for this preprint (which was not certified by peer review) is the author/funder. All rights reserved. No reuse allowed without permission. 1 Karrikin-sensing protein KAI2 is a new player in regulating root growth patterns 2 3 4 Stéphanie M. Swarbreck1*, Yannick Guerringue1,2, Elsa Matthus1, Fiona J. C. Jamieson1,3 and 5 Julia M. Davies1 6 7 1 Department of Plant Sciences, University of Cambridge, Cambridge, CB2 3EA, United 8 Kingdom 9 2 ENS de Lyon - Site Monod, Lyon, 69007, France 10 3 Department of Plant Sciences, University of Oxford, South Parks Road, Oxford, OX1 3RB, 11 United Kingdom 12 * Corresponding author, [email protected], 01223-748-980 13 14 Total word count: 6,192 15 Word count Introduction: 708 16 Word count Materials and Methods: 1,419 17 Word count Results: 2,557 18 Word count Discussion: 1,236 19 Total number of figures: 8 20 Total number of supplementary figures: 6 21 22 1 bioRxiv preprint doi: https://doi.org/10.1101/195891; this version posted September 29, 2017. The copyright holder for this preprint (which was not certified by peer review) is the author/funder. All rights reserved. No reuse allowed without permission. 23 Summary 24 Roots form highly complex systems varying in growth direction and branching pattern to 25 forage for nutrients efficiently. Here mutations in the KAI2 (KARRIKIN INSENSITIVE) a/b- 26 fold hydrolase and the MAX2 (MORE AXILLARY GROWTH 2) F-box leucine-rich protein, 27 which together perceive karrikins (smoke-derived butenolides), caused alteration in root 28 growth direction (root skewing and waving) of Arabidopsis thaliana.