Accreting He-Rich Matter Onto White Dwarfs
Total Page:16
File Type:pdf, Size:1020Kb
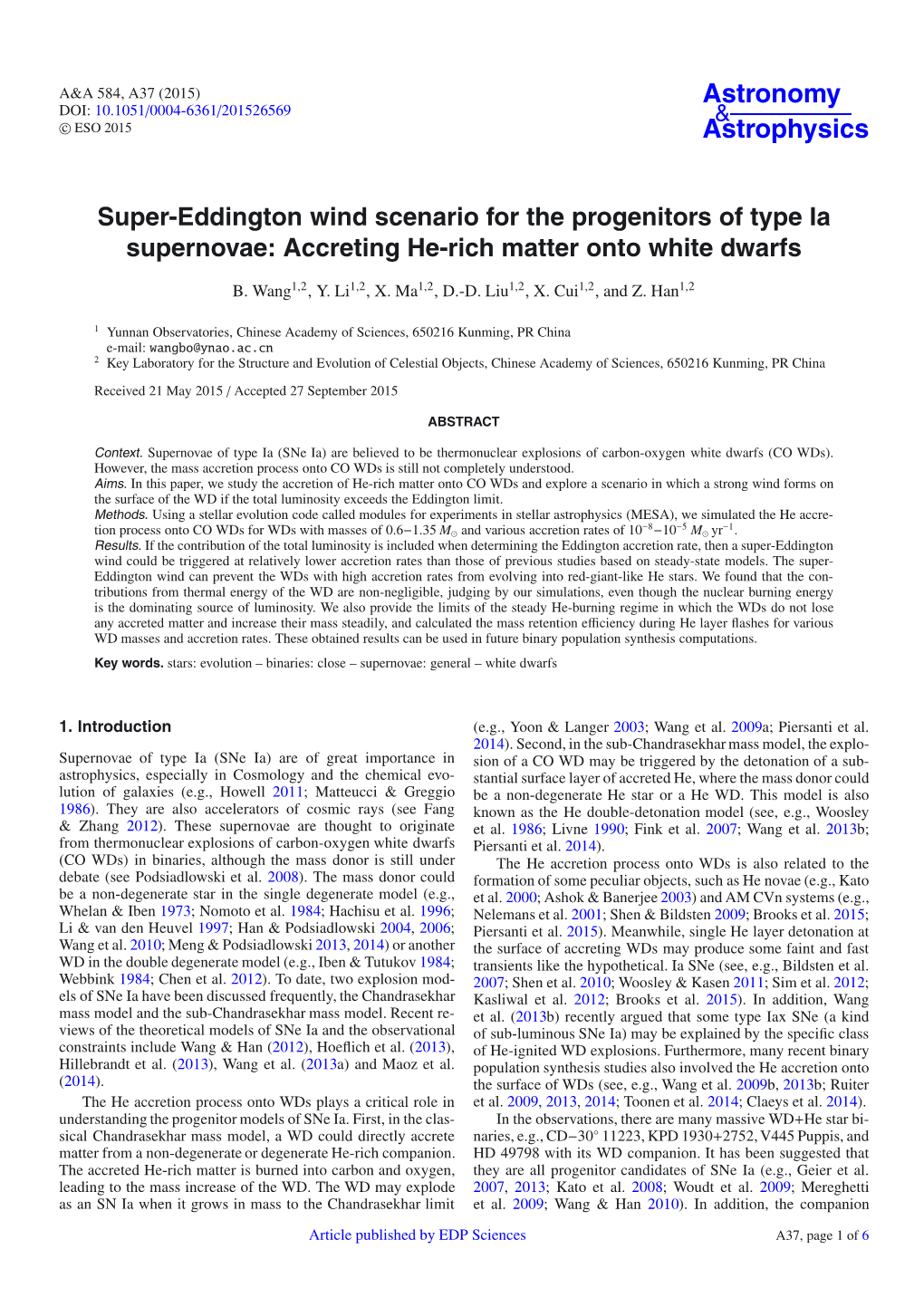
Load more
Recommended publications
-
Arxiv:1403.4250V2 [Astro-Ph.GA] 9 Jun 2014 Nelemans Et Al
Submitted to The Astrophysical Journal Preprint typeset using LATEX style emulateapj v. 5/2/11 CONSTRAINTS ON THE PROGENITOR SYSTEM OF THE TYPE IA SUPERNOVA 2014J FROM PRE-EXPLOSION HUBBLE SPACE TELESCOPE IMAGING Patrick L. Kelly1, Ori D. Fox1, Alexei V. Filippenko1, S. Bradley Cenko2, Lisa Prato3, Gail Schaefer4, Ken J. Shen1,5, WeiKang Zheng1, Melissa L. Graham1, and Brad E. Tucker1,6 Submitted to The Astrophysical Journal ABSTRACT We constrain the properties of the progenitor system of the highly reddened Type Ia supernova (SN) 2014J in Messier 82 (M82; d ≈ 3:5 Mpc). We determine the SN location using Keck-II K-band adaptive optics images, and we find no evidence for flux from a progenitor system in pre-explosion near-ultraviolet through near-infrared Hubble Space Telescope (HST) images. Our upper limits exclude systems having a bright red giant companion, including symbiotic novae with luminosities comparable to that of RS Ophiuchi. While the flux constraints are also inconsistent with predictions for compar- atively cool He-donor systems (T . 35,000 K), we cannot preclude a system similar to V445 Puppis. The progenitor constraints are robust across a wide range of RV and AV values, but significantly greater values than those inferred from the SN light curve and spectrum would yield proportionally brighter luminosity limits. The comparatively faint flux expected from a binary progenitor system consisting of white dwarf stars would not have been detected in the pre-explosion HST imaging. In- frared HST exposures yield more stringent constraints on the luminosities of very cool (T < 3000 K) companion stars than was possible in the case of SN Ia 2011fe. -
Thunderkat: the Meerkat Large Survey Project for Image-Plane Radio Transients
ThunderKAT: The MeerKAT Large Survey Project for Image-Plane Radio Transients Rob Fender1;2∗, Patrick Woudt2∗ E-mail: [email protected], [email protected] Richard Armstrong1;2, Paul Groot3, Vanessa McBride2;4, James Miller-Jones5, Kunal Mooley1, Ben Stappers6, Ralph Wijers7 Michael Bietenholz8;9, Sarah Blyth2, Markus Bottcher10, David Buckley4;11, Phil Charles1;12, Laura Chomiuk13, Deanne Coppejans14, Stéphane Corbel15;16, Mickael Coriat17, Frederic Daigne18, Erwin de Blok2, Heino Falcke3, Julien Girard15, Ian Heywood19, Assaf Horesh20, Jasper Horrell21, Peter Jonker3;22, Tana Joseph4, Atish Kamble23, Christian Knigge12, Elmar Körding3, Marissa Kotze4, Chryssa Kouveliotou24, Christine Lynch25, Tom Maccarone26, Pieter Meintjes27, Simone Migliari28, Tara Murphy25, Takahiro Nagayama29, Gijs Nelemans3, George Nicholson8, Tim O’Brien6, Alida Oodendaal27, Nadeem Oozeer21, Julian Osborne30, Miguel Perez-Torres31, Simon Ratcliffe21, Valerio Ribeiro32, Evert Rol6, Anthony Rushton1, Anna Scaife6, Matthew Schurch2, Greg Sivakoff33, Tim Staley1, Danny Steeghs34, Ian Stewart35, John Swinbank36, Kurt van der Heyden2, Alexander van der Horst24, Brian van Soelen27, Susanna Vergani37, Brian Warner2, Klaas Wiersema30 1 Astrophysics, Department of Physics, University of Oxford, UK 2 Department of Astronomy, University of Cape Town, South Africa 3 Department of Astrophysics, Radboud University, Nijmegen, the Netherlands 4 South African Astronomical Observatory, Cape Town, South Africa 5 ICRAR, Curtin University, Perth, Australia 6 University -
The Orbital Period of V458 Vulpeculae, a Post Double Common-Envelope Nova
Mon. Not. R. Astron. Soc. 000, 1–6 (2010) Printed 11 November 2018 (MN LATEX style file v2.2) The orbital period of V458 Vulpeculae, a post double common-envelope nova P. Rodr´ıguez-Gil1,2,3⋆, M. Santander-Garc´ıa1,2,3, C. Knigge4, R. L. M. Corradi2,3, B. T. G¨ansicke5, M. J. Barlow6, J. J. Drake7, J. Drew8, B. Miszalski8, R. Napiwotzki8, D. Steeghs5, R. Wesson6, A. A. Zijlstra9, D. Jones9, T. Liimets10,1, S. Pyrzas1,5 and M. M. Rubio-D´ıez1,11 1Isaac Newton Group of Telescopes, Apartado de Correos 321, Santa Cruz de La Palma, E-38700, Spain 2Instituto de Astrof´ısica de Canarias, V´ıa L´actea, s/n, La Laguna, E-38205, Santa Cruz de Tenerife, Spain 3Departamento de Astrof´ısica, Universidad de La Laguna, La Laguna, E-38205, Santa Cruz de Tenerife, Spain 4School of Physics and Astronomy, University of Southampton, Southampton SO17 1BJ, UK 5Department of Physics, University of Warwick, Coventry CV4 7AL, UK 6Department of Physics and Astronomy, University College London, Gower Street, London WC1E 6BT, UK 7Harvard-Smithsonian Center for Astrophysics, 60 Garden Street, Cambridge, MA 02138, USA 8Centre for Astrophysics Research, STRI, University of Hertfordshire, College Lane Campus, Hatfield AL10 9AB, UK 9Jodrell Bank Center for Astrophysics, Alan Turing Building, The University of Manchester, Oxford Road, Manchester M13 9PL, UK 10Tartu Observatoorium, T˜oravere 61602, Estonia 11Centro de Astrobiolog´ıa (CSIC/INTA), Ctra. de Torrej´on a Ajalvir, km 4, E-28850 Torrej´on de Ardoz, Madrid, Spain Accepted 2010. Received 2010 ABSTRACT We present time-resolved optical spectroscopy of V458 Vulpeculae (Nova Vul 2007 No. -
Appendix: Spectroscopy of Variable Stars
Appendix: Spectroscopy of Variable Stars As amateur astronomers gain ever-increasing access to professional tools, the science of spectroscopy of variable stars is now within reach of the experienced variable star observer. In this section we shall examine the basic tools used to perform spectroscopy and how to use the data collected in ways that augment our understanding of variable stars. Naturally, this section cannot cover every aspect of this vast subject, and we will concentrate just on the basics of this field so that the observer can come to grips with it. It will be noticed by experienced observers that variable stars often alter their spectral characteristics as they vary in light output. Cepheid variable stars can change from G types to F types during their periods of oscillation, and young variables can change from A to B types or vice versa. Spec troscopy enables observers to monitor these changes if their instrumentation is sensitive enough. However, this is not an easy field of study. It requires patience and dedication and access to resources that most amateurs do not possess. Nevertheless, it is an emerging field, and should the reader wish to get involved with this type of observation know that there are some excellent guides to variable star spectroscopy via the BAA and the AAVSO. Some of the workshops run by Robin Leadbeater of the BAA Variable Star section and others such as Christian Buil are a very good introduction to the field. © Springer Nature Switzerland AG 2018 M. Griffiths, Observer’s Guide to Variable Stars, The Patrick Moore 291 Practical Astronomy Series, https://doi.org/10.1007/978-3-030-00904-5 292 Appendix: Spectroscopy of Variable Stars Spectra, Spectroscopes and Image Acquisition What are spectra, and how are they observed? The spectra we see from stars is the result of the complete output in visible light of the star (in simple terms). -
12899 (Stsci Edit Number: 0, Created: Monday, July 9, 2012 8:35:02 PM EST) - Overview
Proposal 12899 (STScI Edit Number: 0, Created: Monday, July 9, 2012 8:35:02 PM EST) - Overview 12899 - Emission line imaging of the bipolar shell in the Helium Nova V445 Puppis Cycle: 20, Proposal Category: GO (Availability Mode: SUPPORTED) INVESTIGATORS Name Institution E-Mail Dr. Danny Steeghs (PI) (ESA Member) (Contact) The University of Warwick [email protected] Dr. Patrick Woudt (CoI) University of Cape Town [email protected] Dr. Matthew Schurch (CoI) University of Cape Town [email protected] Dr. Margarita Karovska (CoI) (AdminUSPI) Smithsonian Institution Astrophysical Observatory [email protected] Dr. Sumner G. Starrfield (CoI) Arizona State University [email protected] Prof. Thomas R. Marsh (CoI) (ESA Member) The University of Warwick [email protected] Prof. Brian Warner (CoI) University of Cape Town [email protected] Prof. Paul Groot (CoI) (ESA Member) Radboud Universiteit Nijmegen [email protected] VISITS Visit Targets used in Visit Configurations used in Visit Orbits Used Last Orbit Planner Run OP Current with Visit? 01 (1) V-V445-PUP WFC3/UVIS 2 09-Jul-2012 21:34:54.0 yes 2 Total Orbits Used ABSTRACT Explosive detonations on the surfaces of accreting white dwarfs play a key role in establishing viable progenitor pathways towards Type Ia supernova explosions. If the white dwarf is accreting hydrogen deficient material, helium shell-flash driven eruptions can occur on its surface. Thus far only one such helium nova event has been observed in Nova Puppis 2000 = V445 Pup. We have discovered an expanding bipolar shell involving fast moving ejecta of He-rich material surrounding this binary. -
The Orbital Period of V458 Vulpeculae, a Postdouble Commonenvelope Nova
Mon. Not. R. Astron. Soc. 407, L21–L25 (2010) doi:10.1111/j.1745-3933.2010.00895.x The orbital period of V458 Vulpeculae, a post-double common-envelope nova P. Rodr´ıguez-Gil,1,2,3 M. Santander-Garc´ıa,1,2,3 C. Knigge,4 R. L. M. Corradi,2,3 B. T. Gansicke,¨ 5 M. J. Barlow,6 J. J. Drake,7 J. Drew,8 B. Miszalski,8 R. Napiwotzki,8 D. Steeghs,5 R. Wesson,6 A. A. Zijlstra,9 D. Jones,10 T. Liimets,1,9 T. Munoz-Darias,˜ 11 S. Pyrzas1,5 and M. M. Rubio-D´ıez1,12 Downloaded from https://academic.oup.com/mnrasl/article/407/1/L21/1051780 by guest on 28 September 2021 1Isaac Newton Group of Telescopes, Apartado de Correos 321, Santa Cruz de La Palma E-38700, Spain 2Instituto de Astrof´ısica de Canarias, V´ıa Lactea,´ s/n, La Laguna E-38205, Santa Cruz de Tenerife, Spain 3Departamento de Astrof´ısica, Universidad de La Laguna, La Laguna E-38205, Santa Cruz de Tenerife, Spain 4School of Physics and Astronomy, University of Southampton, Southampton SO17 1BJ 5Department of Physics, University of Warwick, Coventry CV4 7AL 6Department of Physics and Astronomy, University College London, Gower Street, London WC1E 6BT 7Harvard–Smithsonian Center for Astrophysics, 60 Garden Street, Cambridge, MA 02138, USA 8Centre for Astrophysics Research, STRI, University of Hertfordshire, College Lane Campus, Hatfield AL10 9AB 9Tartu Observatoorium, Toravere˜ 61602, Estonia 10Jodrell Bank Center for Astrophysics, Alan Turing Building, The University of Manchester, Oxford Road, Manchester M13 9PL 11INAF-Osservatorio Astronomico di Brera, Via E. -
Arxiv:1011.6063V1 [Astro-Ph.SR] 28 Nov 2010 617Russia 369167 00 a on Nteaa Rhv.A Httm,Tebihns O Brightness the Time, That K at by Archive
Peremennye Zvezdy (Variable Stars) 30, No.4, 2010 Received 29 September; accepted 5 October. The progenitor and remnant of the helium nova V445 Puppis V. P. Goranskij1, S. Yu. Shugarov1,2, A. V. Zharova1, P. Kroll3, and E. A. Barsukova4 1 Sternberg Astronomical Institute, Moscow University, Universitetsky Ave. 13, Moscow, 199992 Russia 2 Astronomical Institute of the Slovak Academy of Sciences, Tatranska Lomnica, 05960 Slovakia 3 Sternwarte Sonneberg, Sternwartestrasse 32, Sonneberg, D-96515 Germany 4 Special Astrophysical Observatory, Russian Academy of Sciences, Nizhny Arkhyz, Karachai-Cherkesia, 369167 Russia V445 Pup was a peculiar nova with no hydrogen spectral lines during the outburst. The spectrum contained strong emission lines of carbon, oxygen, calcium, sodium, and iron. We have performed dig- ital processing of photographic images of the V445 Pup progenitor using astronomical plate archives. The brightness of the progenitor in the B band was 14m. 3. It was a periodic variable star, its most probable period being 0d. 650654 ± 0d. 000011. The light curve shape suggests that the progenitor was a common-envelope binary with a spot on the surface and variable surface brightness. The spectral energy distribution of the progenitor between 0.44 and 2.2 µm was similar to that of an A0V type star. After the explosion in 2001, the dust was formed in the ejecta, and the star became a strong infrared source. This resulted in the star’s fading below 20m in the V band. Our CCD BV R observations acquired between 2003 and 2009 suggest that the dust absorption minimum finished in 2004, and the remnant reappeared at the level of 18m. -
Cycle 20 Abstract Catalog (Based on Phase I Submissions)
Cycle 20 Abstract Catalog (Based on Phase I Submissions) Proposal Category: AR Scientific Category: STAR FORMATION ID: 12814 Program Title: Signatures of turbulence in directly imaged protoplanetary disks Principal Investigator: Philip Armitage PI Institution: University of Colorado at Boulder HST imaging of the edge-on protoplanetary disk in the HH 30 system has shown that substantial changes to the morphology of the disk occur on time scales much shorter than the local dynamical time. This may be due to time- variable obscuration of starlight by the turbulent inner disk. More recently, mid-infrared variability of protoplanetary disks has been attributed to a similar physical origin. We propose a theoretical investigation that will establish whether the optical and infrared variability is due to turbulent variations in the scale height of dust in the inner disk, and determine what observations of that variability can tell us of the nature of disk turbulence. We will use a new generation of global magnetohydrodynamic simulations to directly model the turbulence in an annulus of the inner disk, extending from the dust destruction radius out to the radius where turbulence is quenched by poor coupling of the gas to magnetic fields. We will use the output of the MHD simulations as input to Monte Carlo radiative transfer calculations of stellar irradiation of the outer disk. We will use these calculations to produce light curves that show how time variable shadowing from the inner disk affects the outer disk observables: scattered light images and the infrared spectral energy distribution. Proposal Category: GO Scientific Category: COOL STARS ID: 12815 Program Title: Photometry of the Coldest Benchmark Brown Dwarf Principal Investigator: Kevin Luhman PI Institution: The Pennsylvania State University In 2011, we used images from the Spitzer Space Telescope to discover the coldest directly imaged companion to a nearby star (300-345 K). -
Pos(GOLDEN 2017)066 , G , F , R
Hydrodynamic Simulations of Classical Novae: Accretion onto CO White Dwarfs as SN Ia Progenitors S. Starrfield∗ ya, M. Bosea, C. Iliadisb, W. R. Hixc;d, C. E. Woodwarde, R. M. Wagner f ;g, h i J. Jóse , M. Hernanz PoS(GOLDEN 2017)066 aArizona State University- Earth and Space Exploration, Tempe, AZ,USA; b University of North Carolina - Physics and Astronomy, Chapel Hill, NC; cOak Ridge National Laboratory, Oak Ridge, TN; dUniversity of Tennessee, Knoxville, TN; eUniversity of Minnesota, Minneapolis, MN; f Large Binocular Telescope Observatory, Tucson, AZ; gOhio State University, Department of Astronomy, Columbus, OH; hUniversity Polytechnic of Catalunya, Barcelona, Catalunya, Spain; iInstitute of Space Sciences, Barcelona, Catalunya, Spain E-mail: [email protected],[email protected],[email protected], [email protected],[email protected],[email protected],[email protected], [email protected] We report on our continuing studies of Classical Nova explosions by following the evolution of thermonuclear runaways (TNRs) on carbon-oxygen (CO) white dwarfs (WDs). We have varied both the mass of the WD and the composition of the accreted material. Rather than assuming that the material has mixed from the beginning, we now rely on the results of the multidimensional (multi-D) studies of mixing as a consequence of the TNRs in WDs that accreted only Solar matter. The multi-D studies find that mixing with the core occurs after the TNR is well underway and reach enrichment levels in agreement with observations of the ejecta abundances. We report on 3 studies in this paper. First, simulations in which we accrete only Solar matter with NOVA (our 1-D, fully implicit, hydro code). -
SUPPLEMENTARY INFORMATION Doi:10.1038/Nature10646
SUPPLEMENTARY INFORMATION doi:10.1038/nature10646 Supplementary Information Prior limits on SN Ia progenitors from optical data Nine Type Ia supernovae (SNe Ia) with preexisting Hubble Space Telescope (HST) data on their host galaxies have been close enough (within 25 Mpc) to search for a progenitor (Supplementary Table 1). No progenitor system has 31,8,9,10 been found; only upper limits have been possible . Limits range from Mg = −3.9 mag in the case of SN 2004W 31 to MI = −8.3 mag for SN 2003cg . These limits provided only poor constraints; in the case of SN 2006dd and SN 2006mr in NGC 1316, they ruled out normal stars with initial masses greater than 6 M at the tip of the asymptotic giant branch (AGB), young post-AGB stars with initial masses greater than 4 M , and post-red-giant stars with initial 8 masses greater than 9 M . HST data reduction Search for the progenitor system of SN 2011fe We searched the HST data archive and found six datasets that contain the site of SN 2011fe. These include the Ad- vanced Camera for Surveys (ACS) images observed in the F435W, F555W, and F814W filters in program GO–9490 (PI: K. Kuntz) on 2002 Nov. 13, a pair of images observed with the Wide Field Planetary Camera 2 (WFPC2) in the F606W filter in program GO–7909 (PI: S. Casertano) on 1998 May 22, and a Wide Field Camera 3 (WFC3) image observed in the F469N filter in program GO–11635 (PI: M. Shara) on 2010 Apr 8. More details on the available HST data are listed in Supplementary Table 2. -
Non-Biceps Cosmology
Non-Biceps Cosmology Jim Rich SPP-IRFU CEA-Saclay 91191 Gif-sur-Yvette [email protected] May 6, 2014 1404.5633 Dark matter-baryons separation at the lowest mass scale: the Bullet Group We report on the X-ray observation of a strong lensing selected group, SL2S J08544-0121, with a total mass of 2.4pm0.6*1014 Msun which revealed a separation of 12420 kpc between the X-ray emitting collisional gas and the collisionless galaxies and dark matter (DM), traced by strong lensing. This source allows to put an order of magnitude estimate to the upper limit to the interaction cross section of DM of 10 cm2 g1. It is the lowest mass object found to date showing a DM-baryons separation and it reveals that the detection of bullet-like objects is not rare and confined to mergers of massive objects opening the possibility of a statistical detection of DM-baryons separation with future surveys. Jim Rich (IRFU) Non-Biceps Cosmology May 2014 2 / 22 1404.5636 The abundance of Bullet-groups in LCDM We estimate the expected distribution of displacements between the two dominant dark matter (DM) peaks (DM-DM displacements) and between DM and gaseous baryon peak (DM-gas displacements) in dark matter halos with masses larger than 1013 Msun/h. We use as a benchmark the observation of SL2S J08544-0121, which is the lowest mass system (1.01014 Msun/h) observed so far featuring a bi-modal dark matter distribution with a dislocated gas component. We find that (50pm10)percent of the dark matter halos with circular velocities in the range 300 km/s to 700 km/s (groups) show DM-DM displacements equal or larger than 186pm30 kpc/h as observed in SL2S J08544-0121. -
The Enigmatic Outburst of V445 Puppis – a Possible Helium Nova?
A&A 409, 1007–1015 (2003) Astronomy DOI: 10.1051/0004-6361:20031160 & c ESO 2003 Astrophysics The enigmatic outburst of V445 Puppis – A possible helium nova? N.M.AshokandD.P.K.Banerjee Physical Research Laboratory, Navrangpura, Ahmedabad 380 009, India e-mail: [email protected] Received 22 January 2003 / Accepted 16 July 2003 Abstract. JHK spectroscopic and photometric observations are reported for the enigmatic, nova-like, variable V445 Puppis which erupted at the end of 2000. The near-IR spectra are hydrogen-deficient and unusually rich in CI lines. The important CI lines are found to be positioned at 1.133, 1.166, 1.175, 1.189, 1.26 and 1.689 µm. Model calculations for the CI lines are done to make the identification of the lines secure. Photometric data, taken on January 2.90 UT, 2001 shortly after the outburst, show the formation of an optically thin dust shell around V445 Puppis. The temperature and upper limit for the mass of the 9 dust shell are estimated to be 1800 K and Mdust 10− M respectively. A subsequent episode of massive dust formation – ∼ indicated by the observed, deep visual dimming – is also seen in JHK images of early November 2001. V445 Puppis has shown two unusual properties for a nova: (i) the hydrogen-deficiency and He/C enrichment of the object as seen from optical and IR data and (ii) synchrotron radio emission which was detected nearly a year after its outburst by other workers. The strange nature of the object is discussed and it is shown that it is difficult to place it in the known categories of eruptive variables viz.