A Dissertation Entitled Na/K-Atpase Mediates Renal Sodium Handling
Total Page:16
File Type:pdf, Size:1020Kb
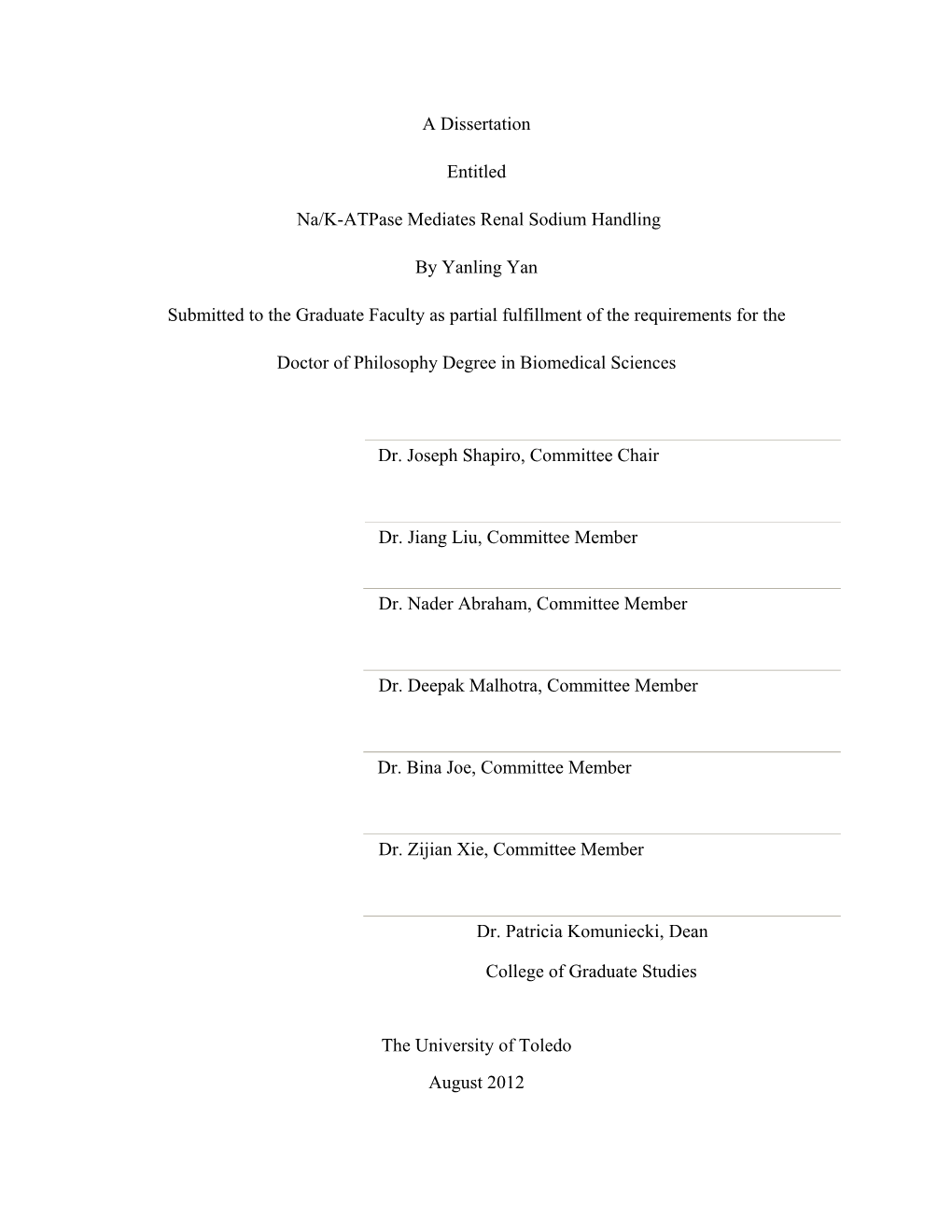
Load more
Recommended publications
-
Ras Gtpase Chemi ELISA Kit Catalog No
Ras GTPase Chemi ELISA Kit Catalog No. 52097 (Version B3) Active Motif North America 1914 Palomar Oaks Way, Suite 150 Carlsbad, California 92008, USA Toll free: 877 222 9543 Telephone: 760 431 1263 Fax: 760 431 1351 Active Motif Europe Waterloo Atrium Drève Richelle 167 – boîte 4 BE-1410 Waterloo, Belgium UK Free Phone: 0800 169 31 47 France Free Phone: 0800 90 99 79 Germany Free Phone: 0800 181 99 10 Telephone: +32 (0)2 653 0001 Fax: +32 (0)2 653 0050 Active Motif Japan Azuma Bldg, 7th Floor 2-21 Ageba-Cho, Shinjuku-Ku Tokyo, 162-0824, Japan Telephone: +81 3 5225 3638 Fax: +81 3 5261 8733 Active Motif China 787 Kangqiao Road Building 10, Suite 202, Pudong District Shanghai, 201315, China Telephone: (86)-21-20926090 Hotline: 400-018-8123 Copyright 2021 Active Motif, Inc. www.activemotif.com Information in this manual is subject to change without notice and does not constitute a commit- ment on the part of Active Motif, Inc. It is supplied on an “as is” basis without any warranty of any kind, either explicit or implied. Information may be changed or updated in this manual at any time. This documentation may not be copied, transferred, reproduced, disclosed, or duplicated, in whole or in part, without the prior written consent of Active Motif, Inc. This documentation is proprietary information and protected by the copyright laws of the United States and interna- tional treaties. The manufacturer of this documentation is Active Motif, Inc. © 2021 Active Motif, Inc., 1914 Palomar Oaks Way, Suite 150; Carlsbad, CA 92008. -
H-Ras Gtpase
H-Ras GTPase Key to Understanding Cancer? Marquette University High School SMART Team: Mohammed Ayesh, Wesley Borden, Andrew Bray, Brian Digiacinto, Patrick Jordan, David Moldenhauer, Thomas Niswonger, Joseph Radke, Amit Singh, Alex Vincent, and Caleb Vogt Teachers: Keith Klestinski and David Vogt Mentor: Evgenii Kovrigin, Ph.D., Medical College of Wisconsin Abstract Cell Cycle Control The protein known as H-Ras GTPase is essential to H-ras is activated late in the G1 phase. proper biological functioning in the entire web of life. The Once H-ras is activated, the cell advances main function of this protein is giving the "stop" signal to past the G1 checkpoint and is compelled to the process of cell reproduction. Unfortunately, this protein complete mitosis. is not perfect and severe consequences, such as cancer, can arise when H-Ras GTPase malfunctions. H-Ras GTPase is a protein from the large family of enzymes that bind and split GTP. H-Ras GTPase is vital in processes like cell-to-cell communication, protein translation in ribosomes, and programmed cell death Ras GTPase Ras GDPase (apoptosis). Its main fields of operation are determining Active Inactive stem cell into specific functioning cells, as well as replicating preexisting "specialized" cells. All G domain based proteins have a universal structure and two Controlling the “Switch” between universal switch mechanisms, which consist of a mixed, six-stranded beta sheet and five alpha helices. H-Ras Active and Inactive States GTPase works by first dissociating from GDP and binding In the graphics (above and below), H-ras is shown in both © 2008, Physiomics, Corp. -
Appendix 2. Significantly Differentially Regulated Genes in Term Compared with Second Trimester Amniotic Fluid Supernatant
Appendix 2. Significantly Differentially Regulated Genes in Term Compared With Second Trimester Amniotic Fluid Supernatant Fold Change in term vs second trimester Amniotic Affymetrix Duplicate Fluid Probe ID probes Symbol Entrez Gene Name 1019.9 217059_at D MUC7 mucin 7, secreted 424.5 211735_x_at D SFTPC surfactant protein C 416.2 206835_at STATH statherin 363.4 214387_x_at D SFTPC surfactant protein C 295.5 205982_x_at D SFTPC surfactant protein C 288.7 1553454_at RPTN repetin solute carrier family 34 (sodium 251.3 204124_at SLC34A2 phosphate), member 2 238.9 206786_at HTN3 histatin 3 161.5 220191_at GKN1 gastrokine 1 152.7 223678_s_at D SFTPA2 surfactant protein A2 130.9 207430_s_at D MSMB microseminoprotein, beta- 99.0 214199_at SFTPD surfactant protein D major histocompatibility complex, class II, 96.5 210982_s_at D HLA-DRA DR alpha 96.5 221133_s_at D CLDN18 claudin 18 94.4 238222_at GKN2 gastrokine 2 93.7 1557961_s_at D LOC100127983 uncharacterized LOC100127983 93.1 229584_at LRRK2 leucine-rich repeat kinase 2 HOXD cluster antisense RNA 1 (non- 88.6 242042_s_at D HOXD-AS1 protein coding) 86.0 205569_at LAMP3 lysosomal-associated membrane protein 3 85.4 232698_at BPIFB2 BPI fold containing family B, member 2 84.4 205979_at SCGB2A1 secretoglobin, family 2A, member 1 84.3 230469_at RTKN2 rhotekin 2 82.2 204130_at HSD11B2 hydroxysteroid (11-beta) dehydrogenase 2 81.9 222242_s_at KLK5 kallikrein-related peptidase 5 77.0 237281_at AKAP14 A kinase (PRKA) anchor protein 14 76.7 1553602_at MUCL1 mucin-like 1 76.3 216359_at D MUC7 mucin 7, -
Small Gtpase Ran and Ran-Binding Proteins
BioMol Concepts, Vol. 3 (2012), pp. 307–318 • Copyright © by Walter de Gruyter • Berlin • Boston. DOI 10.1515/bmc-2011-0068 Review Small GTPase Ran and Ran-binding proteins Masahiro Nagai 1 and Yoshihiro Yoneda 1 – 3, * highly abundant and strongly conserved GTPase encoding ∼ 1 Biomolecular Dynamics Laboratory , Department a 25 kDa protein primarily located in the nucleus (2) . On of Frontier Biosciences, Graduate School of Frontier the one hand, as revealed by a substantial body of work, Biosciences, Osaka University, 1-3 Yamada-oka, Suita, Ran has been found to have widespread functions since Osaka 565-0871 , Japan its initial discovery. Like other small GTPases, Ran func- 2 Department of Biochemistry , Graduate School of Medicine, tions as a molecular switch by binding to either GTP or Osaka University, 2-2 Yamada-oka, Suita, Osaka 565-0871 , GDP. However, Ran possesses only weak GTPase activ- Japan ity, and several well-known ‘ Ran-binding proteins ’ aid in 3 Japan Science and Technology Agency , Core Research for the regulation of the GTPase cycle. Among such partner Evolutional Science and Technology, Osaka University, 1-3 molecules, RCC1 was originally identifi ed as a regulator of Yamada-oka, Suita, Osaka 565-0871 , Japan mitosis in tsBN2, a temperature-sensitive hamster cell line (3) ; RCC1 mediates the conversion of RanGDP to RanGTP * Corresponding author in the nucleus and is mainly associated with chromatin (4) e-mail: [email protected] through its interactions with histones H2A and H2B (5) . On the other hand, the GTP hydrolysis of Ran is stimulated by the Ran GTPase-activating protein (RanGAP) (6) , in con- Abstract junction with Ran-binding protein 1 (RanBP1) and/or the large nucleoporin Ran-binding protein 2 (RanBP2, also Like many other small GTPases, Ran functions in eukaryotic known as Nup358). -
GTP-Binding Proteins • Heterotrimeric G Proteins
GTP-binding proteins • Heterotrimeric G proteins • Small GTPases • Large GTP-binding proteins (e.g. dynamin, guanylate binding proteins, SRP- receptor) GTP-binding proteins Heterotrimeric G proteins Subfamily Members Prototypical effect Gs Gs, Golf cAMP Gi/o Gi, Go, Gz cAMP , K+-current Gq Gq, G11, G14, Inositol trisphosphate, G15/16 diacylglycerol G12/13 G12, G13 Cytoskeleton Transducin Gt, Gustducin cGMP - phosphodiesterase Offermanns 2001, Oncogene GTP-binding proteins Small GTPases Family Members Prototypical effect Ras Ras, Rap, Ral Cell proliferation; Cell adhesion Rho Rho, Rac, CDC42 Cell shape change & motility Arf/Sar Arf, Sar, Arl Vesicles: fission and fusion Rab Rab (1-33) Membrane trafficking between organelles Ran Ran Nuclear membrane plasticity Nuclear import/export The RAB activation-inactivation cycle REP Rab escort protein GGT geranylgeranyl-transferase GDI GDP-dissociation inhibitor GDF GDI displacementfactor Lipid e.g. RAS e.g. ARF1 modification RAB, Gi/o of GTP-binding proteins Lipid modification Enzyme Reaction Myristoylation N-myristoyl-transferase myristoylates N-terminal glycin Farnesylation Farnesyl-transferase, Transfers prenyl from prenyl-PPi Geranylgeranylation geranylgeranyltransferase to C-terminal CAAX motif Palmitoylation DHHC protein Cysteine-S-acylation General scheme of coated vesicle formation T. J. Pucadyil et al., Science 325, 1217-1220 (2009) Published by AAAS General model for scission of coated buds T. J. Pucadyil et al., Science 325, 1217-1220 (2009) Published by AAAS Conformational change in Arf1 and Sar1 GTPases, regulators of coated vesicular transport This happens if protein is trapped in the active conformation Membrane tubules formed by GTP- bound Arf1 Membrane tubules formed by GTP-bound Sar1 Published by AAAS T. -
Small Gtpases of the Ras and Rho Families Switch On/Off Signaling
International Journal of Molecular Sciences Review Small GTPases of the Ras and Rho Families Switch on/off Signaling Pathways in Neurodegenerative Diseases Alazne Arrazola Sastre 1,2, Miriam Luque Montoro 1, Patricia Gálvez-Martín 3,4 , Hadriano M Lacerda 5, Alejandro Lucia 6,7, Francisco Llavero 1,6,* and José Luis Zugaza 1,2,8,* 1 Achucarro Basque Center for Neuroscience, Science Park of the Universidad del País Vasco/Euskal Herriko Unibertsitatea (UPV/EHU), 48940 Leioa, Spain; [email protected] (A.A.S.); [email protected] (M.L.M.) 2 Department of Genetics, Physical Anthropology, and Animal Physiology, Faculty of Science and Technology, UPV/EHU, 48940 Leioa, Spain 3 Department of Pharmacy and Pharmaceutical Technology, Faculty of Pharmacy, University of Granada, 180041 Granada, Spain; [email protected] 4 R&D Human Health, Bioibérica S.A.U., 08950 Barcelona, Spain 5 Three R Labs, Science Park of the UPV/EHU, 48940 Leioa, Spain; [email protected] 6 Faculty of Sport Science, European University of Madrid, 28670 Madrid, Spain; [email protected] 7 Research Institute of the Hospital 12 de Octubre (i+12), 28041 Madrid, Spain 8 IKERBASQUE, Basque Foundation for Science, 48013 Bilbao, Spain * Correspondence: [email protected] (F.L.); [email protected] (J.L.Z.) Received: 25 July 2020; Accepted: 29 August 2020; Published: 31 August 2020 Abstract: Small guanosine triphosphatases (GTPases) of the Ras superfamily are key regulators of many key cellular events such as proliferation, differentiation, cell cycle regulation, migration, or apoptosis. To control these biological responses, GTPases activity is regulated by guanine nucleotide exchange factors (GEFs), GTPase activating proteins (GAPs), and in some small GTPases also guanine nucleotide dissociation inhibitors (GDIs). -
RBD11, a Bioengineered Rab11-Binding Module for Visualizing and Analyzing Endogenous Rab11
bioRxiv preprint doi: https://doi.org/10.1101/2021.01.25.428185; this version posted January 26, 2021. The copyright holder for this preprint (which was not certified by peer review) is the author/funder. All rights reserved. No reuse allowed without permission. RBD11, a bioengineered Rab11-binding module for visualizing and analyzing endogenous Rab11 Futaba Osaki1, Takahide Matsui1,*, Shu Hiragi1, Yuta Homma1, and Mitsunori Fukuda1,2,* 1Laboratory of Membrane Trafficking Mechanisms, Department of Integrative Life Sciences, Graduate School of Life Sciences, Tohoku University, Aobayama, Aoba-ku, Sendai, Miyagi 980-8578, Japan Running title: Development of RBD11, a specific Rab11-binding module 2Lead Contact *Correspondence: Dr. Takahide Matsui, [email protected]; Tel.: +81-22-795-3641; Fax: +81-22-795-3642 Dr. Mitsunori Fukuda, [email protected]; Tel.: +81-22-795-7731; Fax: +81-22-795-7733 1 bioRxiv preprint doi: https://doi.org/10.1101/2021.01.25.428185; this version posted January 26, 2021. The copyright holder for this preprint (which was not certified by peer review) is the author/funder. All rights reserved. No reuse allowed without permission. ABSTRACT The small GTPase Rab11 plays pivotal roles in diverse physiological phenomena, including the recycling of membrane proteins, cytokinesis, neurite outgrowth, and epithelial morphogenesis. One effective method of analyzing the function of endogenous Rab11 is to overexpress a Rab11-binding domain of one of its effectors, e.g., the C-terminal domain of Rab11-FIP2 (Rab11-FIP2-C), as a dominant-negative construct. However, the drawback of this method is the broader Rab binding specificity of the effector domain, because Rab11-FIP2-C binds to Rabs other than Rab11, e.g., to Rab14 and Rab25. -
Subassembly of the Herpes Simplex Virus 1 Helicase-Primase Composed of the UL5 and UL52 Gene Products
Proc. Nati. Acad. Sci. USA Vol. 88, pp. 1105-1109, February 1991 Biochemistry Association of DNA helicase and primase activities with a subassembly of the herpes simplex virus 1 helicase-primase composed of the UL5 and UL52 gene products MARK S. DODSON AND I. R. LEHMAN Department of Biochemistry, Beckman Center, Stanford University School of Medicine, Stanford, CA 94305 Contributed by I. R. Lehman, November 5, 1990 ABSTRACT Herpes simplex virus 1 encodes a helicase- Enzymatic Assays and Gel Electrophoresis. DNA-depen- primase that is composed of the products ofthe UL5, UL8, and dent nucleoside triphosphatase assays were performed as UL52 genes. A stable subassembly consisting of only the UL5 described (2, 8). One unit of enzyme hydrolyzes 1.0 nmol of and UL52 gene products has been purified to near homogeneity nucleoside triphosphate per hr (2). The coupled primase from insect cells doubly infected with baculovirus recombinant assay was used to follow primase activity during purification for these two genes. The purified subassembly has the DNA- of the UL5/UL52 subassembly (3, 9). One unit of primase dependent ATPase, DNA-dependent GTPase, DNA helicase, incorporates 1.0 pmol of [3H]dATP per hr (3). DNA helicase and DNA primase activities that are characteristic of the assays were performed as described (2). The unwound prod- three-subunit holoenzyme. The purified UL8 gene product, ucts were visualized by autoradiography and quantitated by although required for viral DNA replication, neither exhibits scanning with a Molecular Dynamics 300A computing den- these enzymatic activities nor stably associates with either the sitometer. SDS/polyacrylamide gel electrophoresis and sil- UL5 or the UL52 gene product. -
Structural Analysis of the Ras Gtpase Activating Protein Catalytic Domain by Semirandom Mutagenesis: Implications for a Mechanism of Interaction with Ras-GTP1
[CANCERRESEARCH54. 5438-5444, October 15, 1994] Structural Analysis of the Ras GTPase Activating Protein Catalytic Domain by Semirandom Mutagenesis: Implications for a Mechanism of Interaction with Ras-GTP1 Lisa Hettich and Mark Marshall2 Department of Medicine, Division of Hematology/Oncology. Indiana UniversüySchool of Medicine and Waither Oncology Center, Indianapolis. indiana 46202 ABSTRACT region of GAP in the regulation of cytoskeletal structure and cell adhe sion to the extracellular matrix (14). Expression of the GAP NH2- The bovine complementary DNA encoding the catalytic domain of Ras terminal SH2-SH3-SH2 region in Rat-2 fibroblasts resulted in a disrup GTPase activating protein was mutagenized semirandomly using a van tionofactinstressfibersandfocalcontacts.Thiseffectisprobablycaused atlon of the polymerase chain reaction. Sixty-four mutated codons were identified with seventeen of the mutations deleterious to Ras GTPase by the action of the GAP-associated protein, p190, and Rho (15-17). activating function. All of the inactivating single mutations affected the Changes in the cytoskeleton and loss of adhesion are important compo structure of the catalytic fragment as assessed by large decreases in nents of cell growth. soluble protein when expressed in Escherichia coli. Upon exnmlnation of In addition to its potential as a Ras effector molecule, GAP is the Ran binding properties of 10 of the mutants, only 1 was measurably currently the best model for examining GTP-dependent protein inter Impaired for I(as binding and 4 appeared to have increased affinity for actions with Ras. Ras-GTP has been shown to bind to GAP, while Ran. These results demonstrate that Ras binding and GTPase activation Ras-GDP does not (18). -
Small G-Protein They Bind GTP, and “OFF” After the GTP Has Been Hydrolyzed to GDP (Which in Turn Remains Bound)
MONOMERIC G-PROTEINS (GTPases) In addition to heterotrimeric G-proteins, there is a superfamily of small monomeric G-proteins. Their size is around 21 kDa, approximately one half of the average size of the subunits of heterotrimeric proteins. These small proteins also operate as switches, which are “ON” when Small G-protein they bind GTP, and “OFF” after the GTP has been hydrolyzed to GDP (which in turn remains bound). There is a larger family of small GTP-binding switch proteins, related to G Monomeric G-proteins are activated by proteins which induce a conformational change resulting in reduced affinity to GDP, and thus in G-protein : heterotrimeric G proteins GDP release. The general term for such proteins is GEF (guanine nucleotide exchange factor). The GEFs of the small G-proteins are not the activated receptors, but rather proteins downstream the signaling cascade. GEFs vary with regard to their mode of activation and their selectivity for specific monomeric G-proteins. Small GTP-binding proteins include (roles indicated): initiation & elongation factors (protein synthesis). Compared to the subunit of heterotrimeric G-proteins, GTPase activity Ras (growth factor signal cascades). of the monomeric G-proteins is very low in the absence of interference. Rab (vesicle targeting and fusion). However, association with a protein of the GAP (GTPase-activating ARF (forming vesicle coatomer coats). protein) type results in very rapid GTP hydrolysis. Ran (transport of proteins into & out of the nucleus). As in the case of GEFs, the activation state of GAPs can be regulated, and Rho (regulation of actin cytoskeleton) GAPs are selective with regard to the small G-proteins they affect. -
Directing Gene Expression to Gustducin-Positive Taste Receptor Cells
The Journal of Neuroscience, July 15, 1999, 19(14):5802–5809 Directing Gene Expression to Gustducin-Positive Taste Receptor Cells Gwendolyn T. Wong, Luis Ruiz-Avila, and Robert F. Margolskee Howard Hughes Medical Institute, Department of Physiology and Biophysics, The Mount Sinai School of Medicine, New York, New York 10029 We have demonstrated that an 8.4 kb segment (GUS8.4 )from Expression of the lacZ transgene from the GUS8.4 promoter and the upstream region of the mouse a-gustducin gene acts as a of endogenous gustducin was coordinately lost after nerve fully functional promoter to target lacZ transgene expression to section and simultaneously recovered after reinnervation, con- the gustducin-positive subset of taste receptor cells (TRCs). firming the functionality of this promoter. Transgenic expression a The GUS8.4 promoter drove TRC expression of the of rat -gustducin restored responsiveness of gustducin null b-galactosidase marker at high levels and in a developmentally mice to both bitter and sweet compounds, demonstrating the appropriate pattern. The gustducin minimal 1.4 kb promoter utility of the gustducin promoter. (GUS1.4 ) by itself was insufficient to specify TRC expression. We also identified an upstream enhancer from the distal portion Key words: gustducin; taste receptor cells; guanine nucleo- of the murine gustducin gene that, in combination with the tide binding regulatory proteins; gustation; transgenic mice; minimal promoter, specified TRC expression of transgenes. promoter identification; b-galactosidase Current models of taste perception in humans suggest four or five sensory cells of the stomach, duodenum, and pancreas (Ho¨fer et primary taste submodalities: sweet, bitter, salty, sour, and umami al., 1996; Ho¨fer and Drenckhahn, 1998). -
RAB11-Mediated Trafficking and Human Cancers: an Updated Review
biology Review RAB11-Mediated Trafficking and Human Cancers: An Updated Review Elsi Ferro 1,2, Carla Bosia 1,2 and Carlo C. Campa 1,2,* 1 Department of Applied Science and Technology, Politecnico di Torino, 24 Corso Duca degli Abruzzi, 10129 Turin, Italy; [email protected] (E.F.); [email protected] (C.B.) 2 Italian Institute for Genomic Medicine, c/o IRCCS, Str. Prov. le 142, km 3.95, 10060 Candiolo, Italy * Correspondence: [email protected] Simple Summary: The small GTPase RAB11 is a master regulator of both vesicular trafficking and membrane dynamic defining the surface proteome of cellular membranes. As a consequence, the alteration of RAB11 activity induces changes in both the sensory and the transduction apparatuses of cancer cells leading to tumor progression and invasion. Here, we show that this strictly depends on RAB110s ability to control the sorting of signaling receptors from endosomes. Therefore, RAB11 is a potential therapeutic target over which to develop future therapies aimed at dampening the acquisition of aggressive traits by cancer cells. Abstract: Many disorders block and subvert basic cellular processes in order to boost their pro- gression. One protein family that is prone to be altered in human cancers is the small GTPase RAB11 family, the master regulator of vesicular trafficking. RAB11 isoforms function as membrane organizers connecting the transport of cargoes towards the plasma membrane with the assembly of autophagic precursors and the generation of cellular protrusions. These processes dramatically impact normal cell physiology and their alteration significantly affects the survival, progression and metastatization as well as the accumulation of toxic materials of cancer cells.