Sonoluminescence: Making Light of an Unclear Past and Exploring the Path Forward
Total Page:16
File Type:pdf, Size:1020Kb
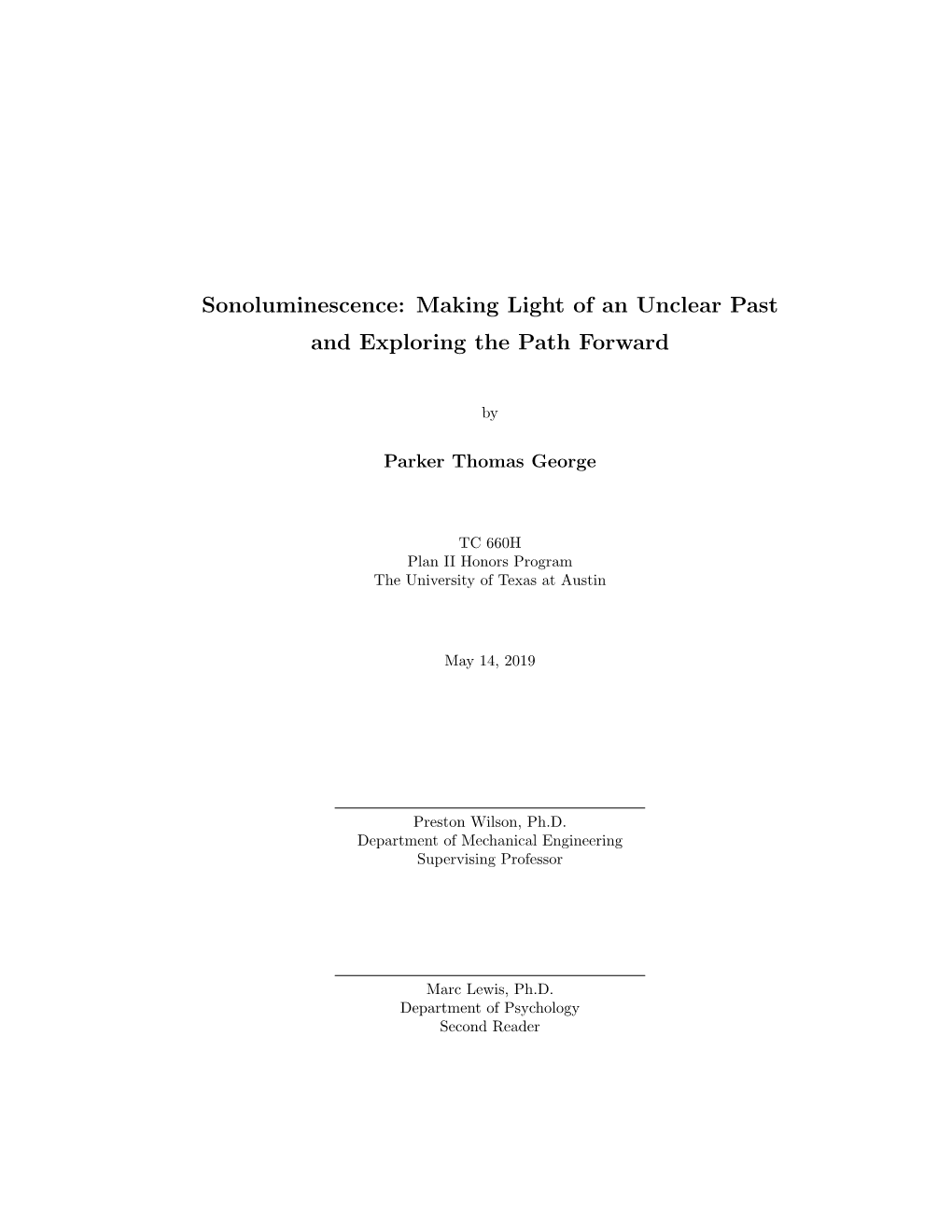
Load more
Recommended publications
-
Modeling the Dynamics of Single-Bubble Sonoluminescence
Modeling the dynamics of single-bubble sonoluminescence Lucas L. Vignoli‡, Ana L. F. de Barros§, Roberto C. A. Thom´ek, A. L. M. A. Nogueira¶, Ricardo C. Paschoal+, and Hil´ario A. Rodrigues∗ Centro Federal de Educa¸c˜ao Tecnol´ogica Celso Suckow da Fonseca – CEFET/RJ Departamento de F´ısica – DEPES Av. Maracan˜a229, 20271-110, Rio de Janeiro, RJ, Brazil Abstract. Sonoluminescence (SL) is the phenomenon in which acoustic energy is (partially) transformed into light. It may occur by means of many or just one bubble of gas inside a liquid medium, giving rise to the terms multi-bubble- and single-bubble sonoluminescence (MBSL and SBSL). In the last years some models have been proposed to explain this phenomenon, but there is still no complete theory for the light emission mechanism (especially in the case of SBSL). In this work, we will not address this more complicated particular issue, but only present a simple model describing the dynamical behaviour of the sonoluminescent bubble, in the SBSL case. Using simple numerical techniques within the software Matlab, we discuss solutions considering various possibilities for some of the parameters involved: liquid compressibility, superficial tension, viscosity, and type of gas. The model may be used as an introductory study of sonoluminescence in physics courses at undergraduate or graduate levels, as well as a quite clarifying example of a physical system exhibiting large nonlinearity. arXiv:1407.5531v1 [physics.ed-ph] 18 Jul 2014 ‡ E-mail: [email protected] § E-mail: [email protected] k E-mail: [email protected] ¶ E-mail: [email protected] + E-mail: [email protected] ∗ E-mail: [email protected] Single bubble sonoluminescence 2 1. -
Recent Cold Fusion Claims: Are They Valid? Ludwik Kowalski Department
Kowalski, L. Recent cold fusion claims: are they valid? in Eleventh International Conference on Condensed Matter Nuclear Science. 2004. Marseille, France. Recent cold fusion claims: are they valid? Ludwik Kowalski Department of Mathematical Sciences Montclair State University, Upper Montclair, NJ, 07043 What is Cold Fusion? Cold fusion (CF) is a mixture of several claims that may or may not be related. Some of them belong to the realm of basic science while others belong to the area of patents. And some seem to be science fiction. From the point of view of history the CF episode, described in several books (1-7) and articles (8,9), is highly unusual. It is a situation in which the validity of research in one particular field has been officially questioned, at least in the US. According to many scientists, the cold fusion claims are in conflict with basic principles of physics and chemistry. That is why most researchers are no longer interested in cold fusion. Surprisingly, however, the field still attracts a large number of investigators with excellent credentials. Once a year they meet at international conferences and publish papers, most often in conference proceedings and over the Internet (10). As a nuclear physicist, and a physics teacher, I examined some of these publications and attended one cold fusion conference (11). My goal here is to describe the main CF claims: (a) unexpected neutrons and protons, (b) unexplained excess heat, and, the most extraordinary, (c) transmutation of chemical elements. The first claim resulted from the work of a physicist, Steven Jones, who wrote about his CF work in the early 1980’s. -
Thermonuclear AB-Reactors for Aerospace
1 Article Micro Thermonuclear Reactor after Ct 9 18 06 AIAA-2006-8104 Micro -Thermonuclear AB-Reactors for Aerospace* Alexander Bolonkin C&R, 1310 Avenue R, #F-6, Brooklyn, NY 11229, USA T/F 718-339-4563, [email protected], [email protected], http://Bolonkin.narod.ru Abstract About fifty years ago, scientists conducted R&D of a thermonuclear reactor that promises a true revolution in the energy industry and, especially, in aerospace. Using such a reactor, aircraft could undertake flights of very long distance and for extended periods and that, of course, decreases a significant cost of aerial transportation, allowing the saving of ever-more expensive imported oil-based fuels. (As of mid-2006, the USA’s DoD has a program to make aircraft fuel from domestic natural gas sources.) The temperature and pressure required for any particular fuel to fuse is known as the Lawson criterion L. Lawson criterion relates to plasma production temperature, plasma density and time. The thermonuclear reaction is realised when L > 1014. There are two main methods of nuclear fusion: inertial confinement fusion (ICF) and magnetic confinement fusion (MCF). Existing thermonuclear reactors are very complex, expensive, large, and heavy. They cannot achieve the Lawson criterion. The author offers several innovations that he first suggested publicly early in 1983 for the AB multi- reflex engine, space propulsion, getting energy from plasma, etc. (see: A. Bolonkin, Non-Rocket Space Launch and Flight, Elsevier, London, 2006, Chapters 12, 3A). It is the micro-thermonuclear AB- Reactors. That is new micro-thermonuclear reactor with very small fuel pellet that uses plasma confinement generated by multi-reflection of laser beam or its own magnetic field. -
Article Thermonuclear Bomb 5 7 12
1 Inexpensive Mini Thermonuclear Reactor By Alexander Bolonkin [email protected] New York, April 2012 2 Article Thermonuclear Reactor 1 26 13 Inexpensive Mini Thermonuclear Reactor By Alexander Bolonkin C&R Co., [email protected] Abstract This proposed design for a mini thermonuclear reactor uses a method based upon a series of important innovations. A cumulative explosion presses a capsule with nuclear fuel up to 100 thousands of atmospheres, the explosive electric generator heats the capsule/pellet up to 100 million degrees and a special capsule and a special cover which keeps these pressure and temperature in capsule up to 0.001 sec. which is sufficient for Lawson criteria for ignition of thermonuclear fuel. Major advantages of these reactors/bombs is its very low cost, dimension, weight and easy production, which does not require a complex industry. The mini thermonuclear bomb can be delivered as a shell by conventional gun (from 155 mm), small civil aircraft, boat or even by an individual. The same method may be used for thermonuclear engine for electric energy plants, ships, aircrafts, tracks and rockets. ----------------------------------------------------------------------- Key words: Thermonuclear mini bomb, thermonuclear reactor, nuclear energy, nuclear engine, nuclear space propulsion. Introduction It is common knowledge that thermonuclear bombs are extremely powerful but very expensive and difficult to produce as it requires a conventional nuclear bomb for ignition. In stark contrast, the Mini Thermonuclear Bomb is very inexpensive. Moreover, in contrast to conventional dangerous radioactive or neutron bombs which generates enormous power, the Mini Thermonuclear Bomb does not have gamma or neutron radiation which, in effect, makes it a ―clean‖ bomb having only the flash and shock wave of a conventional explosive but much more powerful (from 1 ton of TNT and more, for example 100 tons). -
Cavitation-Induced Fusion: Proof of Concept Vol
1 Fomitchev-Zamilov: Cavitation-Induced Fusion: Proof of Concept Vol. 9 Cavitation-Induced Fusion: Proof of Concept Max I. Fomitchev-Zamilov Quantum Potential Corporation, 200 Innovation Blvd, Suite 254, State College, PA 16803 e-mail: [email protected] Cavitation-induced fusion (also known as bubble fusion or sonofusion) has been a topic of much debate and controversy and is generally (albeit incorrectly) perceived as unworkable. In this paper we present the theoretical foundations of cavitation-induced fusion and summarize the experimental results of the research conducted in the past 20 years. Based on the systematic study of all available data we conclude that the cavitation-induced fusion is feasible, doable, and can be used for commercial power generation. We present the results of our own research and disclose a commercial reactor prototype. threatened), to tenure and promotion issues and academic 1. Introduction misconduct (Krivit, 2011). As a result of the ensuing Nuclear fusion (which powers the sun) is the energy of the “bubblegate” scandal Taleyarkhan’s career was destroyed future: 10 microgram of deuterium is equivalent to a barrel of (Reich, 2009) and CIF research became a taboo. oil. Deuterium is cheap, plentiful and easily extracted from What was forgotten amid the outburst of emotions is that water. Unlike uranium fission in modern nuclear power plants cavitation-induced fusion is a fruitful area of research that must be deuterium fusion does not produce radioactive waste. Yet continued: no less than 7 independent peer-reviewed reports despite 40 years of research and over $20B in government exist demonstrating neutron emissions from collapsing spending (Chu, 2008) on inertial/magnetic confinement cavitation bubbles; even heavily criticized experiments by projects (ICF/MCF) the fusion power remains out of reach: to Taleyarkhan’s group have been successfully repeated (Xu & this date there are no fusion reactors capable of sustained Butt, 2005), (Forringer, Robbins, & Martin, 2006), (Bugg, 2006). -
ITER Aims and Technical Challenges
I and I I - ITER Aims and Technical Challenges Jo Lister CRPP-EPFL Centre de Recherches en Physique des Plasmas Ecole Polytechnique Fédérale de Lausanne Switzerland Outline of the first 2 talks q A previous lecture series was given by Prof. Ambrogio Fasoli q These introduced fusion and ITER but concentrated on the physics q Today and tomorrow we revisit the basic aims and design of ITER, but try to extract the technical challenges, and more importantly, where these challenges come from Jo Lister, CRPP-EPFL, "ITER Technical Challenges" CERN, May/June 2005 2 Remember why we are doing this ! q Finite oil resource thinking was launched by Hubbert in 1956 when he predicted US oil production would peak around 1970 - no-one listened q It did - he was right - US production was allowed at 100% capacity q Now domestic production is decreasing q Idem for world oil, idem for gas Provide a long term energy supply Jo Lister, CRPP-EPFL, "ITER Technical Challenges" CERN, May/June 2005 3 We don’t have a solution for demand exceeding production ! q Swenson’s Law says: “ To avoid deprivation resulting from the exhaustion of non-renewable resources, humanity must employ conservation and renewable resource substitutes sufficient to match depletion.” q One reason no-one important wants to know is that oil depletion might (?) lead to war, famine, plague…. Match supply and demand Jo Lister, CRPP-EPFL, "ITER Technical Challenges" CERN, May/June 2005 4 Fusion physics basics - Nuclear ?? q Where does fusion energy come from ? • The nucleus, B/A is the energy “stored” -
Cold Fusion Again 1 Introduction
Prespacetime Journal j February 2016 j Volume 7 j Issue 2 j pp. 379-396 379 Pitk¨anen,M., Cold Fusion Again Exploration Cold Fusion Again Matti Pitk¨anen 1 Abstract During years I have developed two models of cold fusion and in this article these models are combined together. The basic idea of TGD based model of cold is that cold fusion occurs in two steps. First dark nuclei (large heff = n × h) with much lower binding energy than ordinary nuclei are formed at magnetic flux tubes possibly carrying monopole flux. These nuclei can leak out the system along magnetic flux tubes. Under some circumstances these dark nuclei can transform to ordinary nuclei and give rise to detectable fusion products. An essential additional condition is that the dark protons can decay to neutrons rapidly enough by exchanges of dark weak bosons effectively massless below atomic length scale. Also beta decays in which dark W boson decays to dark electron and neutrino can be considered. This allows to overcome the Coulomb wall and explains why final state nuclei are stable and the decay to ordinary nuclei does not yield only protons. Thus it seems that this model combined with the TGD variant of Widom-Larsen model could explain nicely the existing data. In this chapter I will describe the steps leading to the TGD inspired model for cold fusion combining the earlier TGD variant of Widom-Larsen modelwith the model inspired by the TGD inspired model of Pollack's fourth phase of water using as input data findings from laser pulse induced cold fusion discovered by Leif Holmlid and collaborators. -
Disputed Inquiry Clears Bubble-Fusion Engineer
NEWS NATURE|Vol 445|15 February 2007 Disputed inquiry clears bubble-fusion engineer An inquiry has exonerated nuclear engineer several researchers worried that Taleyarkhan’s Rusi Taleyarkhan of misconduct with respect work may be fraudulent, and he wrote to Purdue to allegations made internally at Purdue Uni- about his concerns in June 2006. These include versity in West Lafayette, Indiana, officials the apparent duplication of data between announced last week. But the announcement reports of supposedly independent experi- may raise more questions than it answers: ments4 (first raised by Nature), and a report5 researchers in the field have criticized the uni- that the spectrum of neutrons that Taleyarkhan versity for failing to say whether the inquiry claims to have detected from bubble fusion considered their concerns that the work may exactly matches that of a standard radioactive be fraudulent. source called californium. Taleyarkhan has Taleyarkhan claims to be able to produce since replied that when he measures neutrons fusion by collapsing bubbles in deuterated emitted by californium in his lab, he finds some- liquids. His work promised to improve pros- thing quite unlike what he sees from his fusion pects for developing a clean source of energy, experiments6. But a recent preprint points out but independent scientists have not been able that Taleyarkhan omitted some of the original to replicate the result. The work had been sub- spectral data in his reply, and that the full data ject to several internal allegations of miscon- set still looks like californium7. duct, including the fact that Taleyarkhan cited The university never responded to Suslick’s a paper by his student and postdoc as “inde- concerns. -
CLIMATE CHANGE, DOLPHINS, SPACESHIPS and ANTIMI- CROBIAL RESISTANCE - the IMPACT of BUBBLE ACOUSTICS Timothy G
CLIMATE CHANGE, DOLPHINS, SPACESHIPS AND ANTIMI- CROBIAL RESISTANCE - THE IMPACT OF BUBBLE ACOUSTICS Timothy G. Leighton Institute of Sound and Vibration Research, Faculty of Engineering and the Environment, University of Southampton, Highfield, Southampton SO17 1BJ, UK email: [email protected] Bubbles couple to sound fields to an extraordinary extent, generating and scattering sound, and changing the chemical, physical and biological environments around them when excited to pul- sate by an appropriate sound field. This paper accompanies a plenary lecture, opening with the way that the sound emitted by bubbles, when they are injected into the ocean by breaking waves, helps track the >1 billion tonnes of atmospheric carbon that transfers between atmosphere and ocean annually. However, compared to carbon dioxide, atmospheric methane has at least 20 times the ability, per molecule, to generate ‘greenhouse’ warming. Worldwide there is more than twice the amount of carbon trapped in the seabed in the form of methane hydrate than the amount of carbon worldwide in all other known conventional fossil fuels. Acoustics can track the release of bubbles of seabed methane as this hydrate dissociates in response to increasing ocean tempera- tures, an effect cited by some as a possible climate apocalypse. Continuing the methane theme, this paper discusses the sounds of methane/ethane ‘waterfalls’ on Titan, Saturn’s largest moon, before returning to Earth’s oceans to discuss how whales and dolphins might use the interaction between sound -
Beamed UV Sonoluminescence by Aspherical Air Bubble Collapse Near Liquid-Metal Microparticles Bradley Boyd1,2, Sergey A
www.nature.com/scientificreports OPEN Beamed UV sonoluminescence by aspherical air bubble collapse near liquid-metal microparticles Bradley Boyd1,2, Sergey A. Suslov3, Sid Becker1, Andrew D. Greentree4 & Ivan S. Maksymov5* Irradiation with UV-C band ultraviolet light is one of the most commonly used ways of disinfecting water contaminated by pathogens such as bacteria and viruses. Sonoluminescence, the emission of light from acoustically-induced collapse of air bubbles in water, is an efcient means of generating UV-C light. However, because a spherical bubble collapsing in the bulk of water creates isotropic radiation, the generated UV-C light fuence is insufcient for disinfection. Here we show, based on detailed theoretical modelling and rigorous simulations, that it should be possible to create a UV light beam from aspherical air bubble collapse near a gallium-based liquid-metal microparticle. The beam is perpendicular to the metal surface and is caused by the interaction of sonoluminescence light with UV plasmon modes of the metal. We estimate that such beams can generate fuences exceeding 10 mJ/cm2, which is sufcient to irreversibly inactivate most common pathogens in water with the turbidity of more than 5 Nephelometric Turbidity Units. The ability of UV-C light (200–280 nm) to inactivate bacteria, viruses and protozoa is widely used as an environmentally-friendly, chemical-free and highly efective means of disinfecting and safeguarding water against pathogens responsible for cholera, polio, typhoid, hepatitis and other bacterial, viral and parasitic diseases1. UV-C light inactivates pathogens through absorption of radiation energy by their cellular RNA and DNA prompting the formation of new bonds between adjacent nucleotides. -
Argon Rectification and the Cause of Light Emission in Single-Bubble
VOLUME 88, NUMBER 7 PHYSICAL REVIEW LETTERS 18FEBRUARY 2002 Argon Rectification and the Cause of Light Emission in Single-Bubble Sonoluminescence Brian D. Storey* Franklin W. Olin College of Engineering, Needham, Massachusetts 02492-1245 Andrew J. Szeri† University of California at Berkeley, Berkeley, California 94720-1740 (Received 25 September 2001; published 31 January 2002) In single-bubble sonoluminescence, repeated brief flashes of light are produced in a gas bubble strongly driven by a periodic acoustic field. A startling hypothesis has been made by Lohse and co-workers [Phys. Rev. Lett. 78, 1359 (1997)] that the non-noble gases in an air bubble undergo chemical reaction into soluble products, leaving only argon. In the present work, this dissociation hypothesis is supported by simulations, although the associated temperatures of about 7000 K seem too low for bremsstrahlung, which has been proposed as the dominant light emission mechanism. This suggests that emission from water vapor and its reaction products, heretofore not included, may play an important role. DOI: 10.1103/PhysRevLett.88.074301 PACS numbers: 78.60.Mq, 47.55.Bx, 47.55.Dz, 47.70.Fw In single-bubble sonoluminescence (SBSL), repeated tion hypothesis states that the polyatomic species in an air brief flashes of light are produced in a gas bubble strongly bubble undergo dissociation in the hot interior during vio- driven by a periodic acoustic field. A startling hypothesis lent collapses. The gases are thought to react with water that the non-noble gases in an air bubble undergo chemical vapor to form soluble products, which are taken up by the reaction into soluble products, leaving only argon [1], has liquid water outside the bubble. -
1 Looking Back at Half a Century of Fusion Research Association Euratom-CEA, Centre De
Looking Back at Half a Century of Fusion Research P. STOTT Association Euratom-CEA, Centre de Cadarache, 13108 Saint Paul lez Durance, France. This article gives a short overview of the origins of nuclear fusion and of its development as a potential source of terrestrial energy. 1 Introduction A hundred years ago, at the dawn of the twentieth century, physicists did not understand the source of the Sun‘s energy. Although classical physics had made major advances during the nineteenth century and many people thought that there was little of the physical sciences left to be discovered, they could not explain how the Sun could continue to radiate energy, apparently indefinitely. The law of energy conservation required that there must be an internal energy source equal to that radiated from the Sun‘s surface but the only substantial sources of energy known at that time were wood or coal. The mass of the Sun and the rate at which it radiated energy were known and it was easy to show that if the Sun had started off as a solid lump of coal it would have burnt out in a few thousand years. It was clear that this was much too shortœœthe Sun had to be older than the Earth and, although there was much controversy about the age of the Earth, it was clear that it had to be older than a few thousand years. The realization that the source of energy in the Sun and stars is due to nuclear fusion followed three main steps in the development of science.