Structural and Biochemical Characterization of UDP-Glucose: Glycoprotein Glucosyltransferase
Total Page:16
File Type:pdf, Size:1020Kb
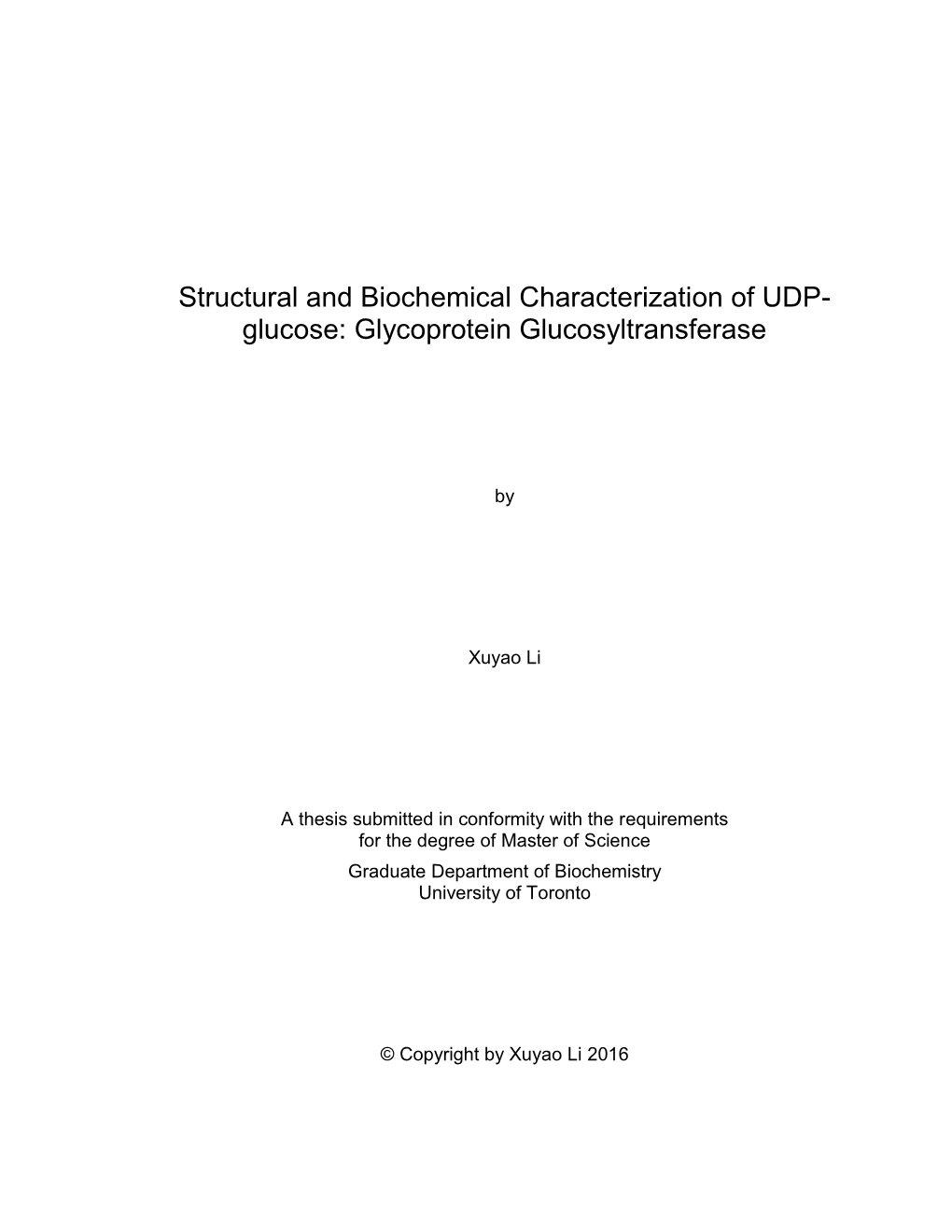
Load more
Recommended publications
-
Protein Folding and Quality Control in the Endoplasmic Reticulum Bertrand Kleizen and Ineke Braakman1
Protein folding and quality control in the endoplasmic reticulum Bertrand Kleizen and Ineke Braakman1 The endoplasmic reticulum (ER) is a highly versatile protein >100 mg/ml. In this gel-like protein matrix, chaperones factory that is equipped with chaperones and folding enzymes and folding enzymes are abundant, greatly outnumbering essential for protein folding. ER quality control guided by the newly synthesised substrates [8]. These folding fac- these chaperones is essential for life. Whereas correctly tors in general prevent aggregation and thereby allow folded proteins are exported from the ER, misfolded proteins more efficient folding of a large variety of proteins. In this are retained and selectively degraded. At least two main review, we highlight the latest advances in understanding chaperone classes, BiP and calnexin/calreticulin, are active how these chaperones and folding enzymes cooperate in in ER quality control. Folding factors usually are found in assisting protein folding and mediating quality control. complexes. Recent work emphasises more than ever that chaperones act in concert with co-factors and with each other. Co-translational and post-translational folding Addresses Mammalian secretory and membrane proteins are synthe- Department of Bio-Organic Chemistry 1, Utrecht University, sised and translocated into the ER by the ribosome/sec61 Padualaan 8, 3584 CH Utrecht, The Netherlands 1 translation/translocation machinery, of which various e-mail: [email protected] enlightening X-ray structures have recently been deter- mined [9,10]. During translation/translocation newly Current Opinion in Cell Biology 2004, 16:343–349 synthesised proteins immediately start to fold. Combin- ing these processes allows sequential folding which may This review comes from a themed issue on greatly enhance folding efficiency, especially of multi- Membranes and organelles Edited by Judith Klumperman and Gillian Griffiths domain proteins [11]. -
The Unfolded Protein Response: an Overview
biology Review The Unfolded Protein Response: An Overview Adam Read 1,2 and Martin Schröder 1,2,* 1 Department of Biosciences, Durham University, South Road, Durham DH1 3LE, UK; [email protected] 2 Biophysical Sciences Institute, Durham University, South Road, Durham DH1 3LE, UK * Correspondence: [email protected]; Tel.: +44-191-334-1316 Simple Summary: The unfolded protein response (UPR) is the cells’ way of maintaining the balance of protein folding in the endoplasmic reticulum, which is the section of the cell designated for folding proteins with specific destinations such as other organelles or to be secreted by the cell. The UPR is activated when unfolded proteins accumulate in the endoplasmic reticulum. This accumulation puts a greater load on the molecules in charge of folding the proteins, and therefore the UPR works to balance this by lowering the number of unfolded proteins present in the cell. This is done in multiple ways, such as lowering the number of proteins that need to be folded; increasing the folding ability of the endoplasmic reticulum and by removing some of the unfolded proteins which take longer to fold. If the UPR is successful at reducing the number of unfolded proteins, the UPR is inactivated and the cells protein folding balance is returned to normal. However, if the UPR is unsuccessful, then this can lead to cell death. Abstract: The unfolded protein response is the mechanism by which cells control endoplasmic reticulum (ER) protein homeostasis. Under normal conditions, the UPR is not activated; however, under certain stresses, such as hypoxia or altered glycosylation, the UPR can be activated due to an accumulation of unfolded proteins. -
Endoplasmic Reticulum-Associated Degradation of Glycoproteins in Plants
MINI REVIEW ARTICLE published: 05 April 2012 doi: 10.3389/fpls.2012.00067 Endoplasmic reticulum-associated degradation of glycoproteins in plants Silvia Hüttner and Richard Strasser* Department of Applied Genetics and Cell Biology, University of Natural Resources and Life Sciences, Vienna, Austria Edited by: In all eukaryotes the endoplasmic reticulum (ER) has a central role in protein folding and Marisa Otegui, University of maturation of secretory and membrane proteins. Upon translocation into the ER polypep- Wisconsin at Madison, USA tides are immediately subjected to folding and modifications involving the formation of Reviewed by: Lorenzo Frigerio, University of disulfide bridges, assembly of subunits to multi-protein complexes, and glycosylation. Dur- Warwick, UK ing these processes incompletely folded, terminally misfolded, and unassembled proteins Federica Brandizzi, Michigan State can accumulate which endanger the cellular homeostasis and subsequently the survival University, USA of cells and tissues. Consequently, organisms have developed a quality control system to *Correspondence: cope with this problem and remove the unwanted protein load from the ER by a process Richard Strasser, Department of Applied Genetics and Cell Biology, collectively referred to as ER-associated degradation (ERAD) pathway. Recent studies in University of Natural Resources and Arabidopsis have identified plant ERAD components involved in the degradation of aber- Life Sciences, Muthgasse 18, 1190 rant proteins and evidence was provided for a specific role -
Interdomain Conformational Flexibility Underpins the Activity of UGGT, the Eukaryotic Glycoprotein Secretion Checkpoint
Interdomain conformational flexibility underpins the activity of UGGT, the eukaryotic glycoprotein secretion checkpoint Pietro Roversia,1,2, Lucia Martib,1, Alessandro T. Caputoa,1, Dominic S. Alonzia,1, Johan C. Hilla,1, Kyle C. Dentc, Abhinav Kumara, Mikail D. Levasseura, Andrea Liaa,b, Thomas Waksmana, Souradeep Basua, Yentli Soto Albrechta, Kristin Qiana, James Patrick McIvora, Colette B. Lippa, Dritan Siliqid, Snezana Vasiljevica, Shabaz Mohammeda, Petra Lukacikc, Martin A. Walshc, Angelo Santinob, and Nicole Zitzmanna,2 aOxford Glycobiology Institute, Department of Biochemistry, University of Oxford, Oxford OX1 3QU, United Kingdom; bInstitute of Sciences of Food Production, Consiglio Nazionale delle Ricerche (CNR) Unit of Lecce, I-73100 Lecce, Italy; cDiamond Light Source and Research Complex at Harwell, Didcot OX11 0DE, United Kingdom; and dIstituto di Cristallografia, Consiglio Nazionale delle Ricerche, I-70126 Bari, Italy Edited by Peter Cresswell, Yale University School of Medicine, New Haven, CT, and approved June 26, 2017 (received for review March 4, 2017) − − Glycoproteins traversing the eukaryotic secretory pathway begin homozygous UGGT1 / knockout of the gene is embryonically life in the endoplasmic reticulum (ER), where their folding is lethal in mice, although cells derived from those embryos are + − surveyed by the 170-kDa UDP-glucose:glycoprotein glucosyltrans- viable (11). More recently, heterozygous UGGT1 / knockout ferase (UGGT). The enzyme acts as the single glycoprotein folding mice have been reported to express approximately half of the quality control checkpoint: it selectively reglucosylates misfolded wild-type (WT) amount of UGGT1 but they undergo normal glycoproteins, promotes their association with ER lectins and development and have no obvious aberrant phenotype (12). associated chaperones, and prevents premature secretion from In healthy cells enjoying steady-state glycoprotein homeosta- the ER. -
UDP-Glucose: Glycoprotein Glucosyltransferase 1 (UGGT1) Substrate Characterization
UDP-glucose: glycoprotein glucosyltransferase 1 (UGGT1) substrate characterization by Nathan Masashi Doner A thesis submitted in conformity with the requirements for the degree of Master of Science Department of Molecular Genetics University of Toronto © Copyright by Nathan Masashi Doner 2018 UDP-glucose: glycoprotein glucosyltransferase 1 (UGGT1) substrate characterization Nathan Doner Master of Science Department of Molecular Genetics University of Toronto 2018 Abstract UDP-glucose: glycoprotein glucosyltransferase 1 (UGGT1) plays a quality control role in the ER by recognizing misfolded glycoproteins and glucosylating their Man9 N-glycans. To study the substrate specificity of UGGT1 and to produce an acceptor substrate for co-crystallization with UGGT1, I have purified Man9-glycosylated forms of bovine RiboB, RiboS, and S-protein. I have also produced variants of these proteins with the single N-glycan at Asn34 re-located to other sites. Using circular dichroism, I have established that RiboB is more thermostable than S-protein and RiboS. Accordingly, S-protein and RiboS are readily glucosylated and bound by human UGGT1. N34Q/Y76N The Man9 form of the S-protein variant bound to UGGT1 with a KD of 76 μM. This substrate is a candidate for co-crystallization with UGGT1. The methods described here can also be used to study other UGGT1 substrates and will facilitate work aimed at understanding how UGGT1 recognizes its misfolded glycoprotein substrates. ii Acknowledgements I would like to thank my supervisor, Dr. James Rini, for being an integral part of my learning process throughout my research. The countless discussions that we had about science, both pertaining to my research, and about other unanswered biological questions, were always stimulating and have contributed to my growth as a scientist. -
A Plant-Specific Calreticulin Is a Key Retention Factor for a Defective Brassinosteroid Receptor in the Endoplasmic Reticulum
A plant-specific calreticulin is a key retention factor for a defective brassinosteroid receptor in the endoplasmic reticulum Hua Jin1, Zhi Hong, Wei Su, and Jianming Li2 Department of Molecular, Cellular, and Developmental Biology, University of Michigan, Ann Arbor, MI 48109-1048 Communicated by Joanne Chory, The Salk Institute for Biological Studies, La Jolla, CA, June 4, 2009 (received for review May 11, 2009) -Mammalian calreticulin (CRT) is a multifunctional Ca2؉-binding mutant suggested that the Arabidopsis CRT1, which can sub protein involved in more than 40 cellular processes in various stitute the mammalian CRT function in cell culture, plays a role .(subcellular compartments, such as Ca2؉ storage and protein fold- in ER stress response (16 ing in the endoplasmic reticulum (ER). CRT homologues were An Arabidopsis dwarf mutant, bri1–9, is an excellent genetic discovered in plants almost 15 years ago, and recent studies system in which to study plant ER quality control (17). BRI1 is revealed that many plant species contain 2 or more CRTs that are a leucine-rich repeat receptor-like kinase that functions as a cell members of 2 distinct families, the CRT1/2 family and the plant- surface receptor for the plant steroid hormone brassinosteroids specific CRT3 family. However, little is known about their physio- (BRs) (18). We have recently discovered that bri1–9, which logical functions. Here we report ebs2 (EMS-mutagenized bri1 carries a S662F mutation in its ligand-binding motif, is retained suppressor 2) as an allele-specific suppressor of bri1–9, a dwarf in the ER by an over-vigilant ER quality control system in Arabidopsis mutant caused by retention of a defective brassino- Arabidopsis (17). -
ER Stress and the Unfolded Protein Response
Mutation Research 569 (2005) 29–63 Review ER stress and the unfolded protein response Martin Schroder¨ a, Randal J. Kaufmanb,∗ a School of Biological and Biomedical Sciences, University of Durham, Durham DH1 3LE, UK b Department of Biological Chemistry and Howard Hughes Medical Institute, University of Michigan Medical Center, 4570 MSRB II, 1150 W. Medical Center Drive, Ann Arbor, MI 48109-0650, USA Received 12 May 2004; accepted 10 June 2004 Abstract Conformational diseases are caused by mutations altering the folding pathway or final conformation of a protein. Many conformational diseases are caused by mutations in secretory proteins and reach from metabolic diseases, e.g. diabetes, to developmental and neurological diseases, e.g. Alzheimer’s disease. Expression of mutant proteins disrupts protein folding in the endoplasmic reticulum (ER), causes ER stress, and activates a signaling network called the unfolded protein response (UPR). The UPR increases the biosynthetic capacity of the secretory pathway through upregulation of ER chaperone and foldase expression. In addition, the UPR decreases the biosynthetic burden of the secretory pathway by downregulating expression of genes encoding secreted proteins. Here we review our current understanding of how an unfolded protein signal is generated, sensed, transmitted across the ER membrane, and how downstream events in this stress response are regulated. We propose a model in which the activity of UPR signaling pathways reflects the biosynthetic activity of the ER. We summarize data that shows that this information is integrated into control of cellular events, which were previously not considered to be under control of ER signaling pathways, e.g. execution of differentiation and starvation programs. -
Protein Folding and Quality Control in the ER
Downloaded from http://cshperspectives.cshlp.org/ on September 25, 2021 - Published by Cold Spring Harbor Laboratory Press Protein Folding and Quality Control in the ER Kazutaka Araki and Kazuhiro Nagata Laboratory of Molecular and Cellular Biology, Faculty of Life Sciences, Kyoto Sangyo University, Kamigamo, Kita-ku, Kyoto 803-8555, Japan Correspondence: [email protected] The endoplasmic reticulum (ER) uses an elaborate surveillance system called the ER quality control (ERQC) system. The ERQC facilitates folding and modification of secretory and mem- brane proteins and eliminates terminally misfolded polypeptides through ER-associated degradation (ERAD) or autophagic degradation. This mechanism of ER protein surveillance is closely linked to redox and calcium homeostasis in the ER, whose balance is presumed to be regulated by a specific cellular compartment. The potential to modulate proteostasis and metabolism with chemical compounds or targeted siRNAs may offer an ideal option for the treatment of disease. he endoplasmic reticulum (ER) serves as a complex in the ER membrane (Johnson and Tprotein-folding factory where elaborate Van Waes 1999; Saraogi and Shan 2011). After quality and quantity control systems monitor arriving at the translocon, translation resumes an efficient and accurate production of secretory in a process called cotranslational translocation and membrane proteins, and constantly main- (Hegde and Kang 2008; Zimmermann et al. tain proper physiological homeostasis in the 2010). Numerous ER-resident chaperones and ER including redox state and calcium balance. enzymes aid in structural and conformational In this article, we present an overview the recent maturation necessary for proper protein fold- progress on the ER quality control system, ing, including signal-peptide cleavage, N-linked mainly focusing on the mammalian system. -
The UDP-Glucose: Glycoprotein Glucosyltransferase (UGGT)
Blanco-Herrera et al. BMC Plant Biology (2015) 15:127 DOI 10.1186/s12870-015-0525-2 RESEARCH ARTICLE Open Access The UDP-glucose: glycoprotein glucosyltransferase (UGGT), a key enzyme in ER quality control, plays a significant role in plant growth as well as biotic and abiotic stress in Arabidopsis thaliana Francisca Blanco-Herrera1, Adrián A. Moreno1,2,RodrigoTapia1, Francisca Reyes1,2, Macarena Araya1,CeciliaD’Alessio3,4, Armando Parodi3 and Ariel Orellana1,2* Abstract Background: UDP-glucose: glycoprotein glucosyltransferase (UGGT) is a key player in the quality control mechanism (ER-QC) that newly synthesized glycoproteins undergo in the ER. It has been shown that the UGGT Arabidopsis orthologue is involved in ER-QC; however, its role in plant physiology remains unclear. Results: Here, we show that two mutant alleles in the At1g71220 locus have none or reduced UGGT activity. In wild type plants, the AtUGGT transcript levels increased upon activation of the unfolded protein response (UPR). Interestingly, mutants in AtUGGT exhibited an endogenous up–regulation of genes that are UPR targets. In addition, mutants in AtUGGT showed a 30 % reduction in the incorporation of UDP-Glucose into the ER suggesting that this enzyme drives the uptake of this substrate for the CNX/CRT cycle. Plants deficient in UGGT exhibited a delayed growth rate of the primary root and rosette as well as an alteration in the number of leaves. These mutants are more sensitive to pathogen attack as well as heat, salt, and UPR-inducing stressors. Additionally, the plants showed impairment in the establishment of systemic acquired resistance (SAR). Conclusions: These results show that a lack of UGGT activity alters plant vegetative development and impairs the response to several abiotic and biotic stresses. -
Glycoprotein Glucosyltransferases in Caenorhabditis and Vertebrates
INVESTIGATION Origin and Evolution of Two Independently Duplicated Genes Encoding UDP- Glucose: Glycoprotein Glucosyltransferases in Caenorhabditis and Vertebrates Diego A. Caraballo,*,2 Lucila I. Buzzi,† Carlos P. Modenutti,‡ Ana Acosta-Montalvo,§ Olga A. Castro,§,1 and María S. Rossi*,1 *CONICET-Universidad de Buenos Aires, IFIBYNE, Buenos Aires, Argentina, Universidad de Buenos Aires, Facultad de Ciencias Exactas y Naturales, Departamento de Fisiología, Biología Molecular y Celular, Buenos Aires, Argentina, †CONICET-Universidad de Buenos Aires, IQUIBICEN, Buenos Aires, Argentina; Universidad de Buenos Aires, Facultad de Ciencias Exactas y Naturales, Departamento de Química Biológica, Buenos Aires, Argentina, ‡CONICET-Universidad de Buenos Aires, IQUIBICEN, Buenos Aires, Argentina; Universidad de Buenos Aires, Facultad de Ciencias Exactas y § Naturales, Departamento de Química Biológica, Buenos Aires, Argentina, and CONICET-Universidad de Buenos Aires, IQUIBICEN, Buenos Aires, Argentina; Universidad de Buenos Aires, Facultad de Ciencias Exactas y Naturales, Departamento de Fisiología y Biología Molecular y Celular, Buenos Aires, Argentina ORCID IDs: 0000-0002-0345-7861 (D.A.C.); 0000-0001-5352-7234 (C.P.M.); 0000-0001-5984-1080 (O.A.C.) ABSTRACT UDP- glucose: glycoprotein glucosyltransferase (UGGT) is a protein that operates as the KEYWORDS gatekeeper for the endoplasmic reticulum (ER) quality control mechanism of glycoprotein folding. It is UDP- glucose: known that vertebrates and Caenorhabditis genomes harbor two uggt gene copies -
ER Quality Control Components UGGT and Stt3a Are Required for Activation of Defense Responses in Bir1-1
RESEARCH ARTICLE ER Quality Control Components UGGT and STT3a Are Required for Activation of Defense Responses in Bir1-1 Qian Zhang, Tongjun Sun, Yuelin Zhang* Department of Botany, University of British Columbia, Vancouver, Canada * [email protected] Abstract The receptor-like kinase SUPPRESSOR OF BIR1, 1 (SOBIR1) functions as a critical regu- lator in plant immunity. It is required for activation of cell death and defense responses in Arabidopsis bak1-interacting receptor-like kinase 1,1 (bir1-1) mutant plants. Here we report that the ER quality control component UDP-glucose:glycoprotein glucosyltransferase (UGGT) is required for the biogenesis of SOBIR1 and mutations in UGGT suppress the OPEN ACCESS spontaneous cell death and constitutive defense responses in bir1-1. Loss of function of Citation: Zhang Q, Sun T, Zhang Y (2015) ER STT3a, which encodes a subunit of the oligosaccharyltransferase complex, also sup- Quality Control Components UGGTand STT3a Are presses the autoimmune phenotype in bir1-1. However, it has no effect on the accumulation Required for Activation of Defense Responses in Bir1-1. PLoS ONE 10(3): e0120245. doi:10.1371/ of SOBIR1, suggesting that additional signaling components other than SOBIR1 may be journal.pone.0120245 regulated by ER quality control. Our study provides clear evidence that ER quality control Academic Editor: Timothy P Devarenne, Texas play critical roles in regulating defense activation in bir1-1. A&M University, UNITED STATES Received: November 17, 2014 Accepted: January 20, 2015 Published: March 16, 2015 Introduction Copyright: © 2015 Zhang et al. This is an open Eukaryotic cells have evolved several quality control mechanisms to monitor the folding of se- access article distributed under the terms of the cretory proteins in the endoplasmic reticulum (ER) [1,2]. -
Arxiv:2008.05608V1 [Physics.Bio-Ph] 12 Aug 2020
Design principles for the glycoprotein quality control pathway Aidan I. Brown1, Elena F. Koslover1*, 1 Department of Physics, University of California, San Diego, San Diego, California 92093 * [email protected] Abstract Newly-translated glycoproteins in the endoplasmic reticulum (ER) often undergo cycles of chaperone binding and release in order to assist in folding. Quality control is required to distinguish between proteins that have completed native folding, those that have yet to fold, and those that have misfolded. Using quantitative modeling, we explore how the design of the quality-control pathway modulates its efficiency. Our results show that an energy-consuming cyclic quality-control process, similar to the observed physiological system, outperforms alternative designs. The kinetic parameters that optimize the performance of this system drastically change with protein production levels, while remaining relatively insensitive to the protein folding rate. Adjusting only the degradation rate, while fixing other parameters, allows the pathway to adapt across a range of protein production levels, aligning with in vivo measurements that implicate the release of degradation-associated enzymes as a rapid-response system for perturbations in protein homeostasis. The quantitative models developed here elucidate design principles for effective glycoprotein quality control in the ER, improving our mechanistic understanding of a system crucial to maintaining cellular health. Author summary We explore the architecture and limitations of the quality-control pathway responsible for efficient folding of secretory proteins. Newly-synthesized proteins are tagged by the attachment of a `glycan' sugar chain which facilitates their binding to a chaperone that assists protein folding. Removal of a specific sugar group on the glycan triggers release from the chaperone, and not-yet-folded proteins can be re-tagged for another round of chaperone binding.