Environmental Effects on Interacting Galaxy Pairs in the SDSS
Total Page:16
File Type:pdf, Size:1020Kb
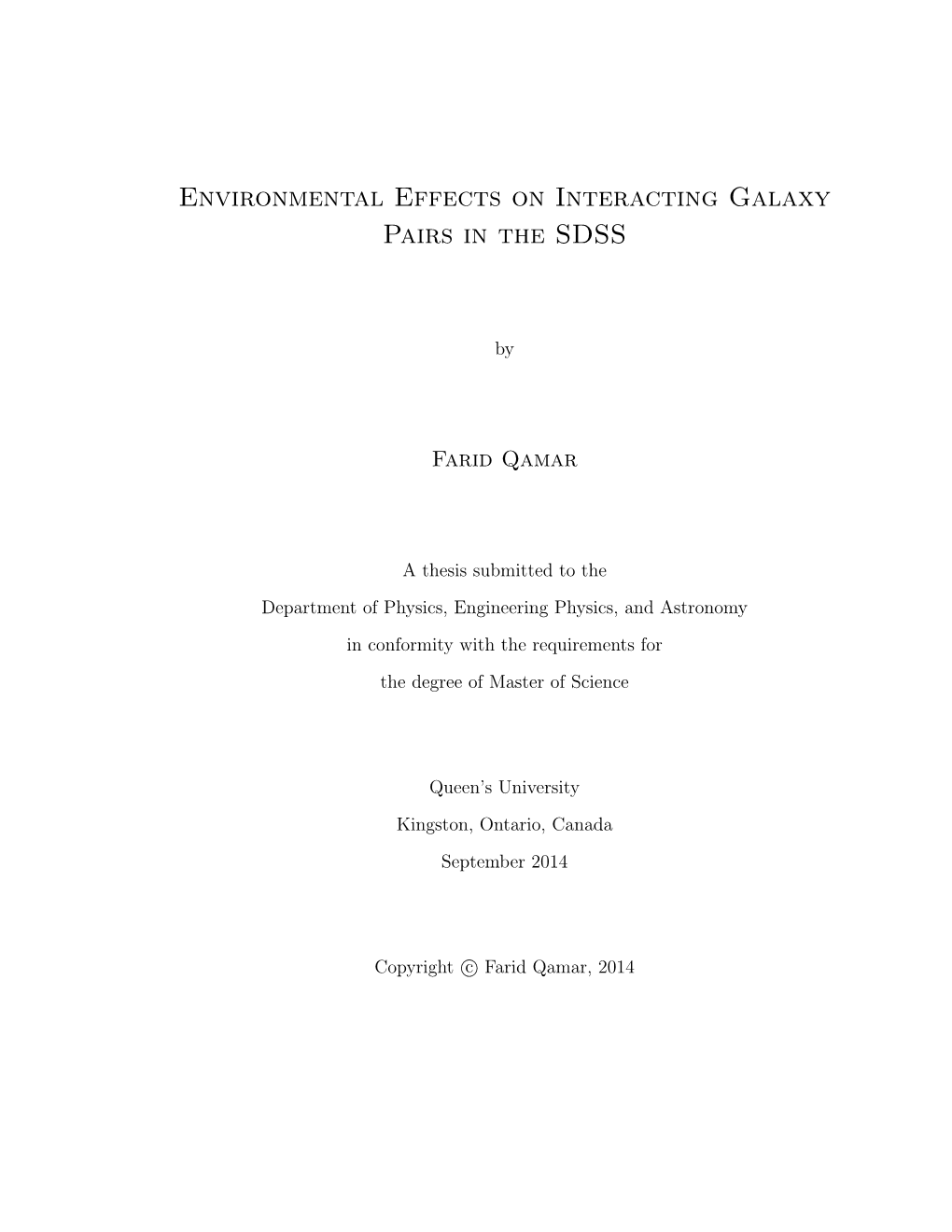
Load more
Recommended publications
-
The Large Scale Universe As a Quasi Quantum White Hole
International Astronomy and Astrophysics Research Journal 3(1): 22-42, 2021; Article no.IAARJ.66092 The Large Scale Universe as a Quasi Quantum White Hole U. V. S. Seshavatharam1*, Eugene Terry Tatum2 and S. Lakshminarayana3 1Honorary Faculty, I-SERVE, Survey no-42, Hitech city, Hyderabad-84,Telangana, India. 2760 Campbell Ln. Ste 106 #161, Bowling Green, KY, USA. 3Department of Nuclear Physics, Andhra University, Visakhapatnam-03, AP, India. Authors’ contributions This work was carried out in collaboration among all authors. Author UVSS designed the study, performed the statistical analysis, wrote the protocol, and wrote the first draft of the manuscript. Authors ETT and SL managed the analyses of the study. All authors read and approved the final manuscript. Article Information Editor(s): (1) Dr. David Garrison, University of Houston-Clear Lake, USA. (2) Professor. Hadia Hassan Selim, National Research Institute of Astronomy and Geophysics, Egypt. Reviewers: (1) Abhishek Kumar Singh, Magadh University, India. (2) Mohsen Lutephy, Azad Islamic university (IAU), Iran. (3) Sie Long Kek, Universiti Tun Hussein Onn Malaysia, Malaysia. (4) N.V.Krishna Prasad, GITAM University, India. (5) Maryam Roushan, University of Mazandaran, Iran. Complete Peer review History: http://www.sdiarticle4.com/review-history/66092 Received 17 January 2021 Original Research Article Accepted 23 March 2021 Published 01 April 2021 ABSTRACT We emphasize the point that, standard model of cosmology is basically a model of classical general relativity and it seems inevitable to have a revision with reference to quantum model of cosmology. Utmost important point to be noted is that, ‘Spin’ is a basic property of quantum mechanics and ‘rotation’ is a very common experience. -
Modeling and Interpretation of the Ultraviolet Spectral Energy Distributions of Primeval Galaxies
Ecole´ Doctorale d'Astronomie et Astrophysique d'^Ile-de-France UNIVERSITE´ PARIS VI - PIERRE & MARIE CURIE DOCTORATE THESIS to obtain the title of Doctor of the University of Pierre & Marie Curie in Astrophysics Presented by Alba Vidal Garc´ıa Modeling and interpretation of the ultraviolet spectral energy distributions of primeval galaxies Thesis Advisor: St´ephane Charlot prepared at Institut d'Astrophysique de Paris, CNRS (UMR 7095), Universit´ePierre & Marie Curie (Paris VI) with financial support from the European Research Council grant `ERC NEOGAL' Composition of the jury Reviewers: Alessandro Bressan - SISSA, Trieste, Italy Rosa Gonzalez´ Delgado - IAA (CSIC), Granada, Spain Advisor: St´ephane Charlot - IAP, Paris, France President: Patrick Boisse´ - IAP, Paris, France Examinators: Jeremy Blaizot - CRAL, Observatoire de Lyon, France Vianney Lebouteiller - CEA, Saclay, France Dedicatoria v Contents Abstract vii R´esum´e ix 1 Introduction 3 1.1 Historical context . .4 1.2 Early epochs of the Universe . .5 1.3 Galaxytypes ......................................6 1.4 Components of a Galaxy . .8 1.4.1 Classification of stars . .9 1.4.2 The ISM: components and phases . .9 1.4.3 Physical processes in the ISM . 12 1.5 Chemical content of a galaxy . 17 1.6 Galaxy spectral energy distributions . 17 1.7 Future observing facilities . 19 1.8 Outline ......................................... 20 2 Modeling spectral energy distributions of galaxies 23 2.1 Stellar emission . 24 2.1.1 Stellar population synthesis codes . 24 2.1.2 Evolutionary tracks . 25 2.1.3 IMF . 29 2.1.4 Stellar spectral libraries . 30 2.2 Absorption and emission in the ISM . 31 2.2.1 Photoionization code: CLOUDY ....................... -
Monthly Newsletter of the Durban Centre - March 2018
Page 1 Monthly Newsletter of the Durban Centre - March 2018 Page 2 Table of Contents Chairman’s Chatter …...…………………….……….………..….…… 3 Andrew Gray …………………………………………...………………. 5 The Hyades Star Cluster …...………………………….…….……….. 6 At the Eye Piece …………………………………………….….…….... 9 The Cover Image - Antennae Nebula …….……………………….. 11 Galaxy - Part 2 ….………………………………..………………….... 13 Self-Taught Astronomer …………………………………..………… 21 The Month Ahead …..…………………...….…….……………..…… 24 Minutes of the Previous Meeting …………………………….……. 25 Public Viewing Roster …………………………….……….…..……. 26 Pre-loved Telescope Equipment …………………………...……… 28 ASSA Symposium 2018 ………………………...……….…......…… 29 Member Submissions Disclaimer: The views expressed in ‘nDaba are solely those of the writer and are not necessarily the views of the Durban Centre, nor the Editor. All images and content is the work of the respective copyright owner Page 3 Chairman’s Chatter By Mike Hadlow Dear Members, The third month of the year is upon us and already the viewing conditions have been more favourable over the last few nights. Let’s hope it continues and we have clear skies and good viewing for the next five or six months. Our February meeting was well attended, with our main speaker being Dr Matt Hilton from the Astrophysics and Cosmology Research Unit at UKZN who gave us an excellent presentation on gravity waves. We really have to be thankful to Dr Hilton from ACRU UKZN for giving us his time to give us presentations and hope that we can maintain our relationship with ACRU and that we can draw other speakers from his colleagues and other research students! Thanks must also go to Debbie Abel and Piet Strauss for their monthly presentations on NASA and the sky for the following month, respectively. -
Arxiv:1802.07727V1 [Astro-Ph.HE] 21 Feb 2018 Tion Systems to Standard Candles in Cosmology (E.G., Wijers Et Al
Astronomy & Astrophysics manuscript no. XSGRB_sample_arxiv c ESO 2018 2018-02-23 The X-shooter GRB afterglow legacy sample (XS-GRB)? J. Selsing1;??, D. Malesani1; 2; 3,y, P. Goldoni4,y, J. P. U. Fynbo1; 2,y, T. Krühler5,y, L. A. Antonelli6,y, M. Arabsalmani7; 8, J. Bolmer5; 9,y, Z. Cano10,y, L. Christensen1, S. Covino11,y, P. D’Avanzo11,y, V. D’Elia12,y, A. De Cia13, A. de Ugarte Postigo1; 10,y, H. Flores14,y, M. Friis15; 16, A. Gomboc17, J. Greiner5, P. Groot18, F. Hammer14, O.E. Hartoog19,y, K. E. Heintz1; 2; 20,y, J. Hjorth1,y, P. Jakobsson20,y, J. Japelj19,y, D. A. Kann10,y, L. Kaper19, C. Ledoux9, G. Leloudas1, A.J. Levan21,y, E. Maiorano22, A. Melandri11,y, B. Milvang-Jensen1; 2, E. Palazzi22, J. T. Palmerio23,y, D. A. Perley24,y, E. Pian22, S. Piranomonte6,y, G. Pugliese19,y, R. Sánchez-Ramírez25,y, S. Savaglio26, P. Schady5, S. Schulze27,y, J. Sollerman28, M. Sparre29,y, G. Tagliaferri11, N. R. Tanvir30,y, C. C. Thöne10, S.D. Vergani14,y, P. Vreeswijk18; 26,y, D. Watson1; 2,y, K. Wiersema21; 30,y, R. Wijers19, D. Xu31,y, and T. Zafar32 (Affiliations can be found after the references) Received/ accepted ABSTRACT In this work we present spectra of all γ-ray burst (GRB) afterglows that have been promptly observed with the X-shooter spectrograph until 31=03=2017. In total, we obtained spectroscopic observations of 103 individual GRBs observed within 48 hours of the GRB trigger. Redshifts have been measured for 97 per cent of these, covering a redshift range from 0.059 to 7.84. -
Using Transfer Learning to Detect Galaxy Mergers
MNRAS 000,1{12 (2017) Preprint 30 May 2018 Compiled using MNRAS LATEX style file v3.0 Using transfer learning to detect galaxy mergers Sandro Ackermann,1 Kevin Schawinski,1 Ce Zhang,2 Anna K. Weigel1 and M. Dennis Turp1 1Institute for Particle Physics and Astrophysics, Department of Physics, ETH Zurich, Wolfgang-Pauli-Strasse 27, CH-8093, Zurich,¨ Switzerland 2Systems Group, Department of Computer Science, ETH Zurich, Universit¨atstrasse 6, CH-8006, Zurich,¨ Switzerland Accepted XXX. Received YYY; in original form ZZZ ABSTRACT We investigate the use of deep convolutional neural networks (deep CNNs) for auto- matic visual detection of galaxy mergers. Moreover, we investigate the use of transfer learning in conjunction with CNNs, by retraining networks first trained on pictures of everyday objects. We test the hypothesis that transfer learning is useful for im- proving classification performance for small training sets. This would make transfer learning useful for finding rare objects in astronomical imaging datasets. We find that these deep learning methods perform significantly better than current state-of-the-art merger detection methods based on nonparametric systems like CAS and GM20. Our method is end-to-end and robust to image noise and distortions; it can be applied directly without image preprocessing. We also find that transfer learning can act as a regulariser in some cases, leading to better overall classification accuracy (p = 0:02). Transfer learning on our full training set leads to a lowered error rate from 0.038±1 down to 0.032±1, a relative improvement of 15%. Finally, we perform a basic sanity- check by creating a merger sample with our method, and comparing with an already existing, manually created merger catalogue in terms of colour-mass distribution and stellar mass function. -
An In-Depth Look at Simulations of Galaxy Interactions a DISSERTATION SUBMITTED to the GRADUATE DIVISION
Theory at a Crossroads: An In-depth Look at Simulations of Galaxy Interactions A DISSERTATION SUBMITTED TO THE GRADUATE DIVISION OF THE UNIVERSITY OF HAWAI‘I AT MANOA¯ IN PARTIAL FULFILLMENT OF THE REQUIREMENTS FOR THE DEGREE OF DOCTOR OF PHILOSOPHY IN ASTRONOMY August 2019 By Kelly Anne Blumenthal Dissertation Committee: J. Barnes, Chairperson L. Hernquist J. Moreno R. Kudritzki B. Tully M. Connelley J. Maricic © Copyright 2019 by Kelly Anne Blumenthal All Rights Reserved ii This dissertation is dedicated to the amazing administrative staff at the Institute for Astronomy: Amy Miyashiro, Karen Toyama, Lauren Toyama, Faye Uyehara, Susan Lemn, Diane Tokumura, and Diane Hockenberry. Thank you for treating us all so well. iii Acknowledgements I would like to thank several people who, over the course of this graduate thesis, have consistently shown me warmth and kindness. First and foremost: my parents, who fostered my love of astronomy, and never doubted that I would reach this point in my career. Larissa Nofi, my partner in crime, has been my perennial cheerleader – thank you for never giving up on me, and for bringing out the best in me. Thank you to David Corbino for always being on my side, and in my ear. To Mary Beth Laychack and Doug Simons: I would not be the person I am now if not for your support. Thank you for trusting me and giving me the space to grow. To my east coast advisor, Lars Hernquist, thank you for hosting me at Harvard and making time for me. To my dear friend, advisor, and colleague Jorge Moreno: you are truly inspirational. -
Tidal Dwarf Galaxies and Missing Baryons
Tidal Dwarf Galaxies and missing baryons Frederic Bournaud CEA Saclay, DSM/IRFU/SAP, F-91191 Gif-Sur-Yvette Cedex, France Abstract Tidal dwarf galaxies form during the interaction, collision or merger of massive spiral galaxies. They can resemble “normal” dwarf galaxies in terms of mass, size, and become dwarf satellites orbiting around their massive progenitor. They nevertheless keep some signatures from their origin, making them interesting targets for cosmological studies. In particular, they should be free from dark matter from a spheroidal halo. Flat rotation curves and high dynamical masses may then indicate the presence of an unseen component, and constrain the properties of the “missing baryons”, known to exist but not directly observed. The number of dwarf galaxies in the Universe is another cosmological problem for which it is important to ascertain if tidal dwarf galaxies formed frequently at high redshift, when the merger rate was high, and many of them survived until today. In this article, I use “dark matter” to refer to the non-baryonic matter, mostly located in large dark halos – i.e., CDM in the standard paradigm. I use “missing baryons” or “dark baryons” to refer to the baryons known to exist but hardly observed at redshift zero, and are a baryonic dark component that is additional to “dark matter”. 1. Introduction: the formation of Tidal Dwarf Galaxies A Tidal Dwarf Galaxy (TDG) is, per definition, a massive, gravitationally bound object of gas and stars, formed during a merger or distant tidal interaction between massive spiral galaxies, and is as massive as a dwarf galaxy [1] (Figure 1). -
Sistema De Control Electrónico Del Espectrógrafo Integral De Campo PUMA Versión 2 (Versión Electrónica, Marzo De 2010) Abel Bernal, Luís A
Sistema de Control Electrónico del Espectrógrafo Integral de Campo PUMA Versión 2 (versión electrónica, marzo de 2010) Abel Bernal, Luís A. Martínez Observatorio Astronómico Nacional-San Pedro Mártir Instituto de Astronomía, Universidad Nacional Autónoma de México Sistema de Control Electrónico Índice Introducción. 2 Descripción Del Sistema. 3 Control Electrónico del Instrumento. 5 Posicionamiento de los Distintos Componentes del PUMA. 5 Control del Paralelismo y la Separación del Fabry-Perot. 6 Descripción General. 8 Control Electrónico del Controlador “Queensgate” CS100. 8 Circuito 1. Manejo de las Líneas Digitales de Control del Fabry-Perot. 9 Circuito 2. Optoacoplamiento para el CS100. 10 Circuito 3. Registros. 11 Control Electrónico del Instrumento. 11 Circuito 4. Control de Motores y Detección de Señales de Posición. 12 Encendido de las Lámparas. 13 Control de los Motores. 13 Circuito 5. Circuito Amplificador para los Motores. 13 Fuentes de voltaje y conexiones. 14 Circuito 6. Fuente de ±12 Volts. 14 El Programa. 15 Descripción del programa. 15 Inicio. 16 El Ciclo central. 17 Las Diferentes Subrutinas, CS100 e Instrumento. 17 Uso Del Sistema. 22 Bibliografía y Agradecimientos. 23 Apéndices. 25 Diagramas y conexiones. 29 Listado de partes. 36 Circuitos Impresos. 37 Listado y lista de variables. 40 Mnemónicos usados en la comunicación. 48 Valores de paralelismo y ajuste de motores. 49 El Convertidor RS232-Ethernet DE-311. 50 1 El PUMA • Introducción El PUMA es un instrumento astronómico para el estudio cinemático de la materia en nebulosas planetarias, regiones de formación estelar, remanentes de supernova y galaxias entre otros [1, 2, 3]. Consiste de un reductor focal 2:1 para longitudes de onda ópticas, que acopla un interferómetro Fabry-Perot de barrido (F-P) [7, 8, 9] al telescopio de 2 m. -
Turbulent Formation of Protogalaxies at the End of the Plasma Epoch: Theory and Observations
Turbulent formation of protogalaxies …, Nova Book, Galaxies: Dynamics, Formation and Evolution 1 Turbulent formation of protogalaxies at the end of the plasma epoch: theory and observations Rudolph E. Schild1,2 1 Center for Astrophysics, 60 Garden Street, Cambridge, MA 02138, USA 2 [email protected] and Carl H. Gibson 3,4 3 University of California San Diego, La Jolla, CA 92093-0411, USA [email protected], http://sdcc3.ucsd.edu/~ir118 ABSTRACT The standard model of gravitational structure formation is based on the Jeans 1902 acoustic theory, neglecting nonlinear instabilities controlled by viscosity, turbulence and diffusion. A linear insta- bility length scale equal to the sound speed times the gravitational freefall time emerges. Because the Jeans scale LJ for the hot primordial plasma is always slightly larger than the scale of causal connection ct, where c is the speed of light and t is the time after the big bang, it has been assumed that no plasma structures could form without guidance from a cold (so LJ CDM is small) collisionless cold-dark-matter CDM fluid to give condensations that gravitationally collect the plasma. Galaxies by this CDM model are therefore produced by gradual hierarchical-clustering of CDM halos to gal- axy mass over billions of years, contrary to observations showing that well formed galaxies existed much earlier. No observations exist of CDM halos. However, Gravitational instability is non- linear and absolute, controlled by viscous and turbulent forces or by diffusivity at Schwarz length scales smaller than ct. Because the universe during the plasma epoch is rapidly expanding, the first structures formed were at density minima by fragmentation when the viscous-gravitional scale LSV first matched ct at 30,000 years to produce protosupercluster voids and protosuperclusters. -
Starburst and Post-Starburst High-Redshift Protogalaxies the Feedback Impact of High Energy Cosmic Rays
A&A 626, A85 (2019) Astronomy https://doi.org/10.1051/0004-6361/201834350 & c ESO 2019 Astrophysics Starburst and post-starburst high-redshift protogalaxies The feedback impact of high energy cosmic rays Ellis R. Owen1,2, Kinwah Wu1,3, Xiangyu Jin4,5, Pooja Surajbali6, and Noriko Kataoka1,7 1 Mullard Space Science Laboratory, University College London, Dorking, Surrey RH5 6NT, UK e-mail: [email protected] 2 Institute of Astronomy, Department of Physics, National Tsing Hua University, Hsinchu, Taiwan (ROC) 3 School of Physics, University of Sydney, Camperdown, NSW 2006, Australia 4 School of Physics, Nanjing University, Nanjing 210023, PR China 5 Department of Physics and McGill Space Institute, McGill University, 3600 University St., Montreal, QC H3A 2T8, Canada 6 Max-Planck-Institut für Kernphysik, Saupfercheckweg 1, Heidelberg 69117, Germany 7 Department of Physics, Faculty of Science, Kyoto Sangyo University, Kyoto 603-8555, Japan Received 1 October 2018 / Accepted 27 April 2019 ABSTRACT Quenching of star-formation has been identified in many starburst and post-starburst galaxies, indicating burst-like star-formation histories (SFH) in the primordial Universe. Galaxies undergoing violent episodes of star-formation are expected to be rich in high energy cosmic rays (CRs). We have investigated the role of these CRs in such environments, particularly how they could contribute to this burst-like SFH via quenching and feedback. These high energy particles interact with the baryon and radiation fields of their host via hadronic processes to produce secondary leptons. The secondary particles then also interact with ambient radiation fields to generate X-rays through inverse-Compton scattering. -
Stars and Gas in the Very Large Interacting Galaxy NGC 6872
A&A 464, 155–165 (2007) Astronomy DOI: 10.1051/0004-6361:20066023 & c ESO 2007 Astrophysics Stars and gas in the very large interacting galaxy NGC 6872 C. Horellou1 and B. Koribalski2 1 Onsala Space Observatory, Chalmers University of Technology, 439 92 Onsala, Sweden e-mail: [email protected] 2 Australia Telescope National Facility, CSIRO, PO Box 76, Epping, NSW 1710, Australia e-mail: [email protected] Received 12 July 2006 / Accepted 2 November 2006 ABSTRACT The dynamical evolution of the large (>100 kpc), barred spiral galaxy NGC 6872 and its small companion IC 4970 in the southern group Pavo is investigated. We present N-body simulations with stars and gas and 21 cm Hi observations carried out with the Australia Telescope Compact Array of the large-scale distribution and kinematics of atomic gas. Hi is detected toward the companion, corre- 9 10 sponding to a gas mass of ∼1.3 × 10 M. NGC 6872 contains ∼1.4 × 10 M of Hi gas, distributed in an extended rotating disk. 9 Massive concentrations of gas (∼10 M) are found at the tip of both tidal tails and towards the break seen in the optical northern arm near the companion. We detect no Hi counterpart to the X-ray trail between NGC 6872 and NGC 6876, the dominant elliptical galaxy in the Pavo group located ∼8 to the southeast. At the sensitivity and the resolution of the observations, there is no sign in the overall Hi distribution that NGC 6876 has affected the evolution of NGC 6872. There is no evidence of ram pressure stripping either. -
A Unified Formation Scenario for the Zoo of Extended Star Clustersand Ultra-Compact Dwarf Galaxies
A UNIFIED FORMATION SCENARIO FOR THE ZOO OF EXTENDED STAR CLUSTERS AND ULTRA-COMPACT DWARF GALAXIES DISSERTATION zur Erlangung des Doktorgrades (Dr. rer. nat.) der Mathematisch-Naturwissenschaftlichen Fakultät der Rheinischen Friedrich-Wilhelms-Universität Bonn vorgelegt von RENATE CLAUDIA BRÜNS aus Bonn Bonn, 2013 Angefertigt mit Genehmigung der Mathematisch-Naturwissenschaftlichen Fakultät der Rheinischen Friedrich-Wilhelms-Universität Bonn 1. Gutachter: Prof. Dr. P. Kroupa 2. Gutachter: Prof. Dr. U. Klein Tag der Promotion: 16. Juni 2014 Erscheinungsjahr: 2014 Contents Abstract 1 1 Introduction 3 1.1 Old Star Clusters in the Local Group ......................... 3 1.2 Old Star Clusters beyond the Local Group ..................... 7 1.3 Ultra-Compact Dwarf Galaxies ............................ 8 1.4 Young Massive Star Clusters and Star Cluster Complexes ............. 9 1.5 Outline of Thesis .................................... 14 2 NumericalMethod 17 2.1 N-BodyCodes ..................................... 17 2.1.1 Set-Up of the Initial Conditions ....................... 18 2.1.2 The Integrator ................................. 22 2.1.3 Determination of Accelerations from the N Particles ............ 23 2.1.4 The Analytical External Tidal Field ..................... 26 2.1.5 Determination of the Enclosed Mass and the Effective Radius ...... 29 2.2 The Particle-Mesh Code SUPERBOX ......................... 29 2.2.1 Definition of the Grids and the Determination of Accelerations ...... 30 2.2.2 Illustration of the Combination of the Grid Potentials ........... 32 2.2.3 General Framework for the Choice of Parameters for the Simulations . 34 2.2.4 SUPERBOX++ versus Fortran SUPERBOX ................... 40 3 ACatalogofECsandUCDsinVariousEnvironments 43 3.1 Introduction ...................................... 43 3.2 The Observational Basis of the EO Catalog ..................... 44 3.2.1 EOs in Late-Type Galaxies .........................