Beta-Delayed Neutron Data and Models for SCALE
Total Page:16
File Type:pdf, Size:1020Kb
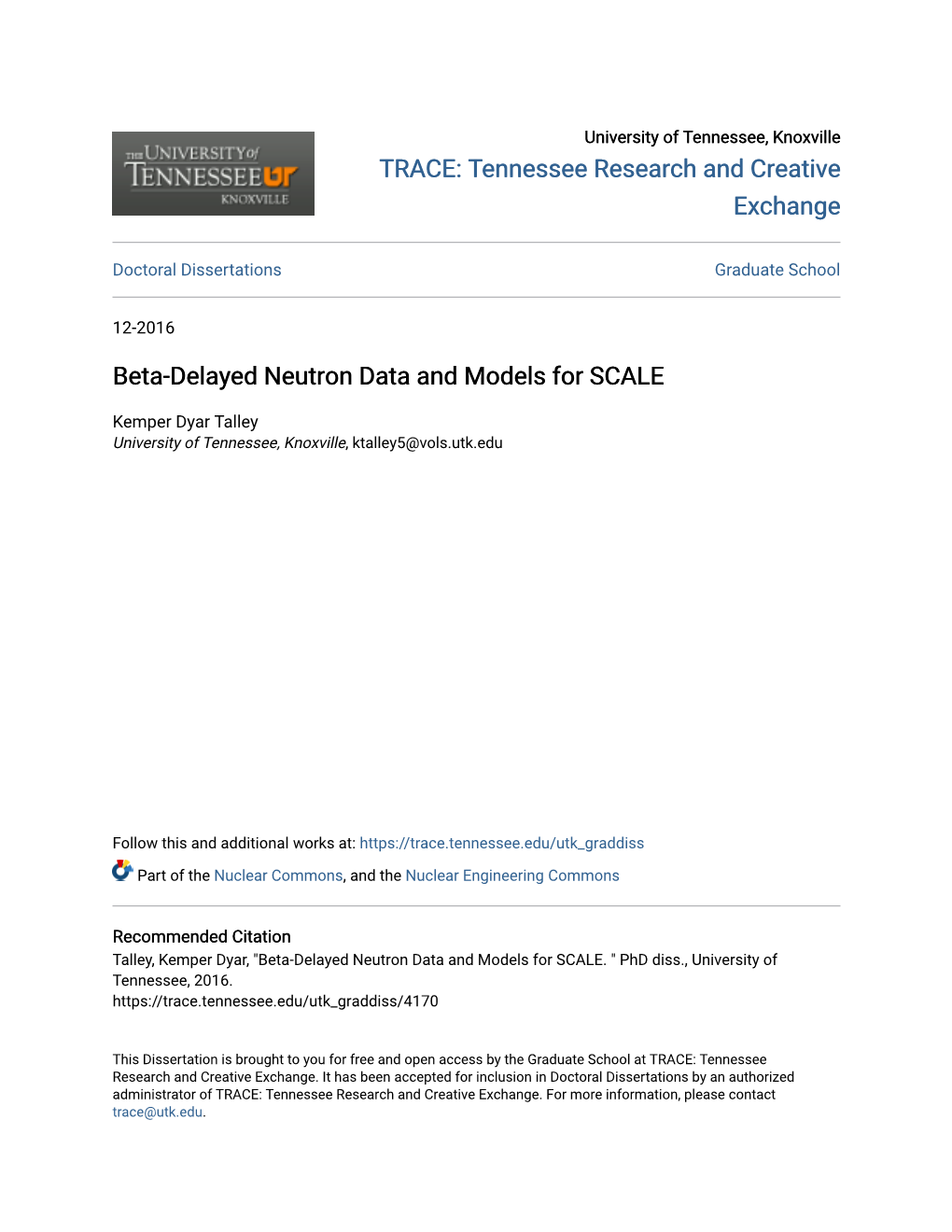
Load more
Recommended publications
-
SCALE 5.1 Predictions of PWR Spent Nuclear Fuel Isotopic Compositions
ORNL/TM-2010/44 SCALE 5.1 Predictions of PWR Spent Nuclear Fuel Isotopic Compositions March 2010 Prepared by G. Radulescu I. C. Gauld G. Ilas DOCUMENT AVAILABILITY Reports produced after January 1, 1996, are generally available free via the U.S. Department of Energy (DOE) Information Bridge: Web site: http://www.osti.gov/bridge Reports produced before January 1, 1996, may be purchased by members of the public from the following source: National Technical Information Service 5285 Port Royal Road Springfield, VA 22161 Telephone: 703-605-6000 (1-800-553-6847) TDD: 703-487-4639 Fax: 703-605-6900 E-mail: [email protected] Web site: http://www.ntis.gov/support/ordernowabout.htm Reports are available to DOE employees, DOE contractors, Energy Technology Data Exchange (ETDE) representatives, and International Nuclear Information System (INIS) representatives from the following source: Office of Scientific and Technical Information P.O. Box 62 Oak Ridge, TN 37831 Telephone: 865-576-8401 Fax: 865-576-5728 E-mail: [email protected] Web site: http://www.osti.gov/contact.html This report was prepared as an account of work sponsored by an agency of the United States Government. Neither the United States government nor any agency thereof, nor any of their employees, makes any warranty, express or implied, or assumes any legal liability or responsibility for the accuracy, completeness, or usefulness of any information, apparatus, product, or process disclosed, or represents that its use would not infringe privately owned rights. Reference herein to any specific commercial product, process, or service by trade name, trademark, manufacturer, or otherwise, does not necessarily constitute or imply its endorsement, recommendation, or favoring by the United States Government or any agency thereof. -
Systematic of Delayed Neutron Parameters
RU0110988 40 XIV International Workshop on Nuclear Fission Physics Systematic of Delayed Neutron Parameters S.G. Isaev, V.M. Piksaikin Institute of Physics and Power Engineering, Obninsk, Russia Abstract The experimental studies of the energy dependence of the delayed neutron parameters for various fissioning systems has shown that the behavior of a some combination of delayed neutron parameters has a similar features. On the basis of this findings the systematics of delayed neutron experimental data for thorium, uranium, plutonium and americium isotopes have been investigated with the purpose to find a correlation of DN parameters with characteristics of fissioning system as well as a correlation between the delayed neutron parameters themselves. Below we will present the preliminary results which were obtained during this study omitting the physics interpretation of the results. Introduction The knowledge of the time dependence of P-delayed neutron emission is of great importance for the development of reliable data base required both for reactor-physics analysis and for the investigation of nuclei which are far from the valley of stability. Since discovery of delayed neutrons the time dependence of delayed neutron emission from neutron induced fission was studied in more than 238 experiments [1]. In parallel to the experimental studies of aggregate DN emission from neutron induced fission the group parameters of delayed neutron emission were calculated using microscopic approach (summation technique) [2]. These calculations are based on the knowledge of the emission probabilities values Pn, half-life values and yields of individual precursors of delayed neutrons [3]. One of the main results of the aggregate DN decay curve measurements was that the group periods (or decay constants) for various fissioning systems for fast neutrons induced fission do not differ from each other [4]. -
System Studies of Fission-Fusion Hybrid Molten Salt Reactors
University of Tennessee, Knoxville TRACE: Tennessee Research and Creative Exchange Doctoral Dissertations Graduate School 12-2013 SYSTEM STUDIES OF FISSION-FUSION HYBRID MOLTEN SALT REACTORS Robert D. Woolley University of Tennessee - Knoxville, [email protected] Follow this and additional works at: https://trace.tennessee.edu/utk_graddiss Part of the Nuclear Engineering Commons Recommended Citation Woolley, Robert D., "SYSTEM STUDIES OF FISSION-FUSION HYBRID MOLTEN SALT REACTORS. " PhD diss., University of Tennessee, 2013. https://trace.tennessee.edu/utk_graddiss/2628 This Dissertation is brought to you for free and open access by the Graduate School at TRACE: Tennessee Research and Creative Exchange. It has been accepted for inclusion in Doctoral Dissertations by an authorized administrator of TRACE: Tennessee Research and Creative Exchange. For more information, please contact [email protected]. To the Graduate Council: I am submitting herewith a dissertation written by Robert D. Woolley entitled "SYSTEM STUDIES OF FISSION-FUSION HYBRID MOLTEN SALT REACTORS." I have examined the final electronic copy of this dissertation for form and content and recommend that it be accepted in partial fulfillment of the equirr ements for the degree of Doctor of Philosophy, with a major in Nuclear Engineering. Laurence F. Miller, Major Professor We have read this dissertation and recommend its acceptance: Ronald E. Pevey, Arthur E. Ruggles, Robert M. Counce Accepted for the Council: Carolyn R. Hodges Vice Provost and Dean of the Graduate School (Original signatures are on file with official studentecor r ds.) SYSTEM STUDIES OF FISSION-FUSION HYBRID MOLTEN SALT REACTORS A Dissertation Presented for the Doctor of Philosophy Degree The University of Tennessee, Knoxville Robert D. -
Controlled Artificial Nucleosyntheses and Electron Nuclear Reactor
International Journal of Engineering and Technical Research (IJETR) ISSN: 2321-0869 (O) 2454-4698 (P) Volume-8, Issue-12, December 2018 Controlled Artificial Nucleosyntheses and Electron Nuclear Reactor Alexander Bolonkin Abstract— By now, about 3000 nuclides are known, which Radionuclides are produced in stellar nucleosynthesis and decay and pass into each other. Many of them can be obtained in supernova explosions along with stable nuclides. Secondary nuclear reactors. The author offers a method for searching for radionuclides are radiogenic isotopes derived from the decay the desired nuclides and a simple controlled method for of primordial radionuclides. Cosmogenic isotopes, such as obtaining artificial nuclides, such that in the chain of subsequent carbon-14, are present because they are continually being decays they contain an alpha decay. With alpha decay, a large amount of nuclear energy is generated that can easily be formed in the atmosphere due to cosmic rays. converted into electricity and reactive traction. This method is Radionuclides are produced as an unavoidable result of simple and safe, does not require large, expensive laser, nuclear fission and thermonuclear explosions. The process of magnetic installations, million temperatures, it can be used in nuclear fission creates a wide range of fission products, most small and medium engines for cars, airplanes, rockets, space of which are radionuclides. Further radionuclides can be vehicles and for unlimited energy on Earth created from irradiation of the nuclear fuel (creating a range of actinides) and of the surrounding structures, yielding Index Terms— controlled nucleosyntheses, artificial activation products. This complex mixture of radionuclides nucleosyntheses, electron nuclear reactor. with different chemistries and radioactivity makes handling nuclear waste and dealing with nuclear fallout particularly problematic. -
Observables of Interest for the Characterisation of Spent Nuclear Fuel
Observables of interest for the characterisation of Spent Nuclear Fuel Gašper Žerovnik Peter Schillebeeckx Kevin Govers Alessandro Borella Dušan Ćalić Luca Fiorito Bor Kos Alexey Stankovskiy Gert Van den Eynde Marc Verwerft 2018 EUR 29301 EN This publication is a Technical report by the Joint Research Centre (JRC), the European Commission’s science and knowledge service. It aims to provide evidence-based scientific support to the European policymaking process. The scientific output expressed does not imply a policy position of the European Commission. Neither the European Commission nor any person acting on behalf of the Commission is responsible for the use that might be made of this publication. Contact information Name: Peter Schillebeeckx Address: Joint Research Center, Retieseweg 111, 2440 Geel, Belgium Email: [email protected] Tel.: +32 (0)14 571 475 JRC Science Hub https://ec.europa.eu/jrc JRC112361 EUR 29301 EN PDF ISBN 978-92-79-90347-2 ISSN 1831-9424 doi:10.2760/418524 Luxembourg: Publications Office of the European Union, 2018 © European Atomic Energy Community, 2018 Reuse is authorised provided the source is acknowledged. The reuse policy of European Commission documents is regulated by Decision 2011/833/EU (OJ L 330, 14.12.2011, p. 39). For any use or reproduction of photos or other material that is not under the EU copyright, permission must be sought directly from the copyright holders. How to cite this report: G. Žerovnik et al., Observables of interest for the characterization of Spent Nuclear Fuel , EUR 29301 EN, Publications Office of the European Union, Luxembourg, 2018, ISBN 978-92-79-90347-2, doi 10.2760/418524, PUBSY No JRC112361. -
Determination of Beta Activity in Water
Determination of Beta Activity in Water 3 Q 3 GEOLOGICAL SURVEY WATER-SUPPLY PAPER 1696-A Determination of Beta Activity in Water By F. B. BARKER and B. P. ROBINSON RADIOCHEMICAL ANALYSIS OF WATER GEOLOGICAL SURVEY WATER-SUPPLY PAPER 1696-A UNITED STATES GOVERNMENT PRINTING OFFICE, WASHINGTON : 1963 UNITED STATES DEPARTMENT OF THE INTERIOR STEWART L. UDALL, Secretary GEOLOGICAL SURVEY Thomas B. Nolan, Director For sale by the Superintendent of Documents, U.S. Government Printing Office Washington 25, D.C. CONTENTS Fag* Abstract__--__-______---____________________-_--____--_--____-_ Al Introduction. ______ _______________________________ ___ 1 Sources of radioactivity in water______________________________ 2 Collecting and preserving the sample_________________________ 5 Measurement of radioactivity______________________ 7 Techniques and instruments._____________________________ 8 Statistical considerations___________________________________ 9 Calibration of instruments____________-__-__-_-____-______-_ 13 Control of instrument operation-_-__--_-__-__---_------_-__--_-_ 16 Optimum operation of G-M counters_______________________ 17 Optimum operation of scintillation and proportional counters. __ 18 Background control chart_________________________________ 19 Standard control chart__-______-_____-__________--______-__ 21 Determination of gross beta activity in water.________________________ 22 Principle of determination___________-________.-._______ 23 Apparatus____________________________________________________ 24 Reagents-________-____-_--__-___________________--___-__-_. -
1- TOPIC: 292001 KNOWLEDGE: K1.02 [3.0/3.1] QID: B45 the Term “Neutron Generation Time” Is Defined As the Average Time Betw
NRC Generic Fundamentals Examination Question Bank--BWR May 2020 TOPIC: 292001 KNOWLEDGE: K1.02 [3.0/3.1] QID: B45 The term “neutron generation time” is defined as the average time between... A. neutron absorption and the resulting fission. B. the production of a delayed neutron and subsequent neutron thermalization. C. neutron absorption producing a fission and absorption or leakage of resultant neutrons. D. neutron thermalization and subsequent neutron absorption. ANSWER: C. TOPIC: 292001 KNOWLEDGE: K1.02 [3.0/3.1] QID: B174 Which one of the following is the definition of the term, prompt neutron? A. A high-energy neutron emitted from a neutron precursor, immediately after the fission process. B. A neutron with an energy level greater than 0.1 MeV, emitted in less than 1.0 x 10-4 seconds following a nuclear fission. C. A neutron emitted in less than 1.0 x 10-14 seconds following a nuclear fission. D. A neutron emitted as a result of a gamma-neutron or alpha-neutron reaction. ANSWER: C. -1- Neutrons NRC Generic Fundamentals Examination Question Bank--BWR May 2020 TOPIC: 292001 KNOWLEDGE: K1.02 [3.0/3.1] QID: B245 Delayed neutrons are neutrons that... A. have reached thermal equilibrium with the surrounding medium. B. are expelled within 1.0 x 10-14 seconds of the fission event. C. are expelled with the lowest average kinetic energy of all fission neutrons. D. are responsible for the majority of U-235 fissions. ANSWER: C. TOPIC: 292001 KNOWLEDGE: K1.02 [3.0/3.1] QID: B1146 (P1945) Which one of the following types of neutrons has an average neutron generation lifetime of 12.5 seconds? A. -
Current Status of Spallation Product Data. Nuclear Engineering View-Point
JP0450476 JAERI-Conf 2004-005 2.4 Current Status of Spallation Product Data: Nuclear Engineering View-Point VArtisyuk, M.Saito, T.Sawada Research Laboratory for Nuclear Reactors, Tokyo Institute of Technology 2-12-1 0-okayama, Meguro-ku, Tokyo 152-8550, Japan TEL: 81-3-5734-3074 FAX: 81-3-5734-2959 e-inail: artisy11k(iDnr.titech.acJV Abstract Proton induced isotopic transformation in spallation targets of accelerator-driven systems (ADS) is becoming a key factor in selecting the reference target material&design options. The present paper gives an outlook of the current status of data on spallation products from the view- point of nuclear egineering stressing the isotopes whose accumulation would significantly effect the target performance. 1. INTRODUCTION The maturing of the accelerator technology toward practical implementation in the form of accelerator-driven systems gave rise to the specific concern about isotope transformation in neutron producing targets. Associated terminology sometimes looks confusing. The term "spallation" that in nuclear physics is generally reserved for identification of specific nuclear reaction with emission of secondary nucleons, by engineering community is taken in a complex like "spallation neutron source" or "spallation target" to identify the vital design element responsible for neutron generation induced by accelerated ions. Strictly speaking, in this element pure spallation reaction might be accompanied by reactions like, for example, high energy fission and multi-fragmentation having a different mechanism from classical spallation In addition to that, if the target is large enough, the secondary nucleons emitted through spallation will be moderated and trigger the nuclear reactions trough mechanism of compound nucleus, like capture reaction. -
(I) CORRELATION PROPERTIES of DELAYED NEUTRONS from FAST NEUTRON INDUCED FISSION
XA9848517-/? International Atomic Energy Agency INDC(CCP)-415 Distr. CI + Special INTERNATIONAL NUCLEAR DATA COMMITTEE TWO REPORTS: (i) CORRELATION PROPERTIES OF DELAYED NEUTRONS FROM FAST NEUTRON INDUCED FISSION V.M. Piksaikin, S.G. Isaev Institute of Physics and Power Engineering, Obninsk, Russia (ii) METHOD AND SET-UP FOR MEASUREMENTS OF TRACE LEVEL CONTENT OF HEAVY FISSIONABLE ELEMENTS BASED ON DELAYED NEUTRON COUNTING V.M. Piksaikin, A.A. Goverdovski, G.M Pshakin Institute of Physics and Power Engineering, Obninsk, Russia October 1998 IAEA NUCLEAR DATA SECTION, WAGRAMERSTRASSE 5, A-1400 VIENNA 29-49 Printed by the IAEA in Austria October 1998 INDC(CCP)-415 Distr. CI + Special TWO REPORTS: (i) CORRELATION PROPERTIES OF DELAYED NEUTRONS FROM FAST NEUTRON INDUCED FISSION V.M. Piksaikin, S.G. Isaev Institute of Physics and Power Engineering, Obninsk, Russia Abstract The experimental studies of the energy dependence of the delayed neutron parameters for various fissioning systems has shown that the behavior of a some combination of delayed neutron parameters (group relative abundances a, and half lives T,) has a similar features. On the basis of this findings the systematics of delayed neutron experimental data for thorium, uranium, plutonium and americium isotopes have been investigated with the purpose to find a correlation of DN parameters with characteristics of fissioning system as well as a correlation between the delayed neutron parameters themselves. Below we will present the preliminary results which were obtained during this study omitting the physics interpretation of the results. (ii) METHOD AND SET-UP FOR MEASUREMENTS OF TRACE LEVEL CONTENT OF HEAVY FISSIONABLE ELEMENTS BASED ON DELAYED NEUTRON COUNTING V.M. -
JAERI-Data/Code 99-008 DEVELOPMENT of the DCHAIN
JAERI-Data/Code JP9950120 99-008 DEVELOPMENT OF THE DCHAIN-SP CODE FOR ANALYZING DECAY AND BUILD-UP CHARACTERISTICS OF SPALLATION PRODUCTS March 1999 Hiroshi TAKADA and Kazuaki KOSAKO* B * if. =§• 13 w n p/f Japan Atomic Energy Research Institute "i*- Mi, 9- (T319-H95 This report is issued irregularly. Inquiries about availability of the reports should be addressed to Research Information Division, Department of Intellectual Resources, Japan Atomic Energy Research Institute, Tokai-mura, Naka-gun, Ibaraki-ken, 319-1195, Japan. ©Japan Atomic Energy Research Institute, 1999 JAERI-Data/Code 99-008 Development of the DCHAIN-SP Code for Analyzing Decay and Build-up Characteristics of Spallation Products Hiroshi TAKADA and Kazuaki KOSAKO* Center for Neutron Science Tokai Research Establishment Japan Atomic Energy Research Institute Tokai-mura, Naka-gun, Ibaraki-ken (Received February 2, 1999) For analyzing the decay and build-up characteristics of spallation products, the DCHAIN-SP code has been developed on the basis of the DCHAIN-2 code by revising the decay data and implementing the neutron cross section data. The decay data are newly processed from the data libraries of EAF 3.1, FENDL/D-1 and ENSDF. The neutron cross section data taken from FENDL/A-2 data library are also prepared to take account of the transmutation of nuclides by the neutron field at the produced position. The DCHAIN-SP code solves the time evolution of decay and build-up of nuclides in every decay chain by the Beteman method. The code can estimate the following physical quantities of produced nuclides : inventory, activity, decay heat by the emission of a , j3 and y -rays, and y -ray energy spectrum, where the nuclide production rate estimated by the nucleon-meson transport code such as NMTC/JAERI97 is used as an input data. -
Beta-Decay Studies for Applied and Basic Nuclear Physics
EPJ manuscript No. (will be inserted by the editor) Beta-decay studies for applied and basic nuclear physics A. Algora1;2, J. L. Tain1, B. Rubio1, M. Fallot3, and W. Gelletly4 1 IFIC (CSIC-Univ. Valencia), Paterna, Spain 2 Institute of Nuclear Research (ATOMKI), Debrecen, Hungary 3 Subatech (CNRS/in2p3 - Univ. Nantes - IMTA), Nantes, France 4 University of Surrey, Surrey, UK Received: date / Revised version: date Abstract. In this review we will present the results of recent beta-decay studies using the total absorption technique that cover topics of interest for applications, nuclear structure and astrophysics. The decays studied were selected primarily because they have a large impact on the prediction of a) the decay heat in reactors, important for the safety of present and future reactors and b) the reactor electron anti- neutrino spectrum, of interest for particle/nuclear physics and reactor monitoring. For these studies the total absorption technique was chosen, since it is the only method that allows one to obtain beta decay probabilities free from a systematic error called the Pandemonium effect. The total absorption technique is based on the detection of the gamma cascades that follow the initial beta decay. For this reason the technique requires the use of calorimeters with very high gamma detection efficiency. The measurements presented and discussed here were performed mainly at the IGISOL facility of the University of Jyv¨askyl¨a (Finland) using isotopically pure beams provided by the JYFLTRAP Penning trap. Examples are presented to show that the results of our measurements on selected nuclei have had a large impact on predictions of both the decay heat and the anti-neutrino spectrum from reactors. -
The Study of Beta-Delayed Neutron Decay Near the Neutron Drip Line
THE STUDY OF BETA-DELAYED NEUTRON DECAY NEAR THE NEUTRON DRIP LINE By Chandana Sujeewa Sumithrarachchi A DISSERTATION Submitted to Michigan State University in partial ful¯llment of the requirements for the degree of DOCTOR OF PHILOSOPHY Department of Chemistry 2007 ABSTRACT The Study of Beta-delayed Neutron Decay Near The Neutron Drip Line By Chandana Sujeewa Sumithrarachchi The study of neutron-rich oxygen and uorine isotopes can provide important information on the evolution of nuclear shell structure close to the neutron drip line. The structural changes in this region are reected with observations of the rapid change in the location of the drip line at uorine and appearance of a new shell closure at N = 14. The recent experiments along with the shell model calculations provide evidence for the doubly magic nature of 22O. The negative parity states in 22O rooted in the neutron pf orbitals are not experimentally known. The knowledge of nuclear structure in 23F, which has the structure of a single proton outside the doubly magic 22O, is also important as it should be sensitive to the proton s and d orbital splitting. The present work focused on the beta-delayed neutron and gamma- ray spectroscopes from 22N and 23O beta decay. The measurements of 22N and 23O were carried out at the NSCL using fragments from the reaction of 48Ca beam in a Be target. The desired isotopes were stopped in the implantation detector and then monitored for beta-delayed neutrons and gamma- rays using a neutron spectroscopic array and eight detectors from SeGA, respectively.