Diptera: Muscidae) and Their
Total Page:16
File Type:pdf, Size:1020Kb
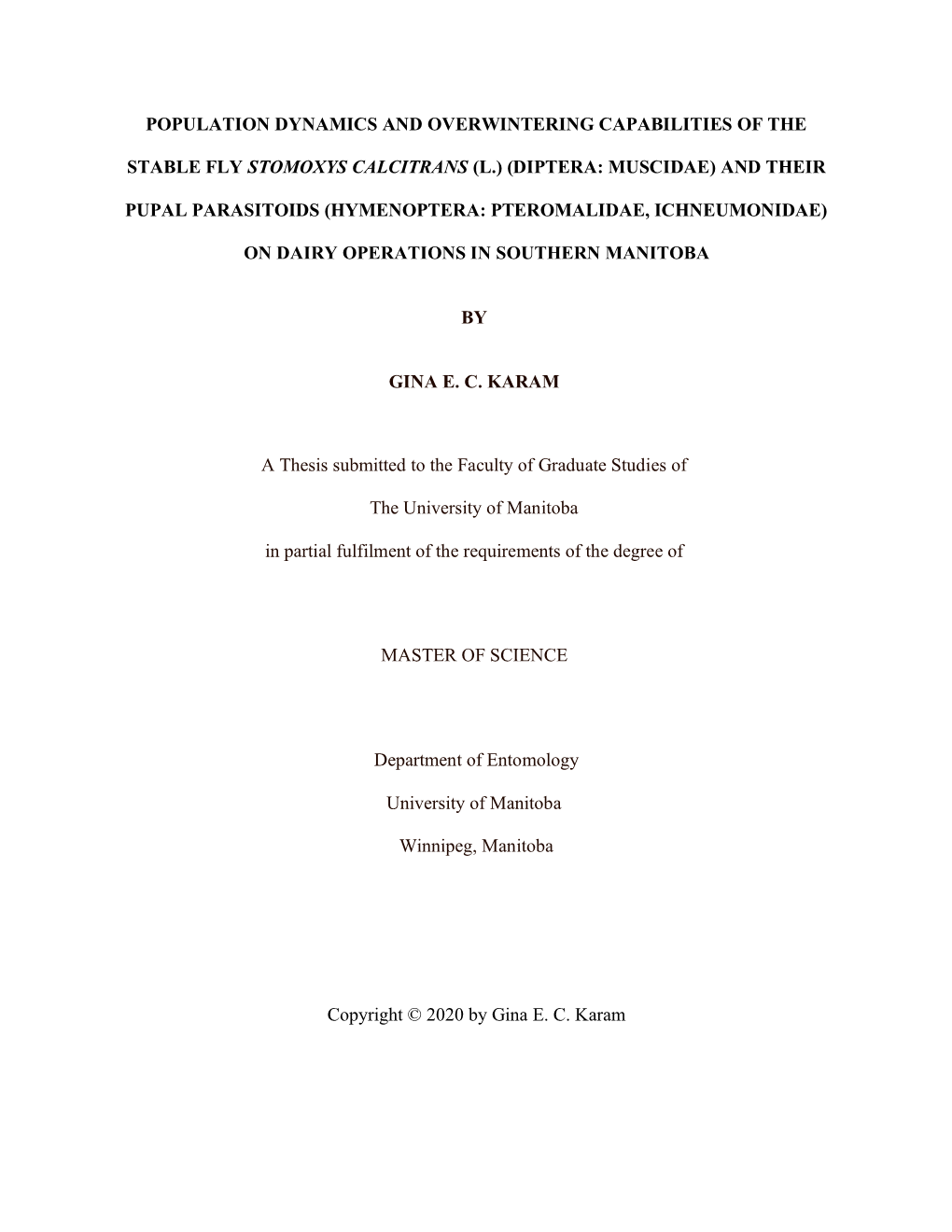
Load more
Recommended publications
-
Diptera: Muscidae) Due to Habronema Muscae (Nematoda: Habronematidae
©2017 Institute of Parasitology, SAS, Košice DOI 10.1515/helm-2017-0029 HELMINTHOLOGIA, 54, 3: 225 – 230, 2017 Preimaginal mortality of Musca domestica (Diptera: Muscidae) due to Habronema muscae (Nematoda: Habronematidae) R. K. SCHUSTER Central Veterinary Research Laboratory, PO Box 597, Dubai, United Arab Emirates, E-mail: [email protected] Article info Summary Received December 29, 2016 In order to study the damage of Habronema muscae (Carter, 1861) on its intermediate host, Mus- Accepted April 24, 2017 ca domestica Linnaeus, 1758, fl y larval feeding experiments were carried out. For this, a defi ned number of praeimaginal stages of M. domestica was transferred in daily intervals (from day 0 to day 10) on faecal samples of a naturally infected horse harboring 269 adult H. muscae in its stomach. The development of M. domestica was monitored until imagines appeared. Harvested pupae were measured and weighted and the success of infection was studied by counting 3rd stage nematode larvae in freshly hatched fl ies. In addition, time of pupation and duration of the whole development of the fl ies was noticed. Pupation, hatching and preimaginal mortality rates were calculated and the number of nematode larvae in freshly hatched fl ies was counted. Adult fl ies harboured up to 60 Habronema larvae. Lower pupal volumes and weights, lower pupation rates and higher preimaginal mortality rates were found in experimental groups with long exposure to parasite eggs compared to experimental groups with short exposure or to the uninfected control groups. Maggots of the former groups pupated earlier and fl y imagines occurred earlier. These fi ndings clearly showed a negative impact of H. -
Ophthalmic and Cutaneous
ISRAEL JOURNAL OF VETERINARY MEDICINE OPHTHALMIC AND CUTANEOUS HABRONEMIASIS IN A HORSE: CASE REPORT AND REVIEW OF THE LITERATURE Yarmut Y., Brommer H., Weisler S., Shelah M., Komarovsky O., and Steinman A*. a Koret School of Veterinary Medicine, Faculty of Agricultural, Food and Environmental Quality Sciences, The Hebrew University of Jerusalem, P.O. Box 12, Rehovot 76100, Israel. b Department of Equine Sciences, Faculty of Veterinary Medicine, Utrecht University. Yalelaan 114, NL-3584 CM, Utrecht, The Netherlands. c Kfar Shmuel 13, 99788, Israel. * Corresponding author. A. Steinman Tel.: +972-54-8820-516; Fax: +972-3-9604-079. E-mail address: [email protected] Hospital (KSVM-VTH). The horse presented skin lesions around INTRODUCTION the medial canthus of the right eye and on the lateral bulb of Habronemiasis is a parasitic disease of equids (horses, donkeysth,e heel of the right front leg. The lesions were first noticed 3 mules and zebras) caused by the nematodes Habronema musca,week s previously and the referring veterinarian had suspected H. majus andDraschia microstoma (1,2). The adult worms livhabronemiasise . The horse was treated with ivermectin 1.87 % on the wall of the stomach of the host without internal migrationper .os (Eqvalan Veterinary® 200 ug/kg, Merial B.V., Haarlem, Embryonated eggs are excreted in the feces to the environmenNetherlands)t , and dexamethasone intramuscularly (Dexacort where they are ingested by the larvae of intermediate hosts, sucForte®h , 20 mg/ml Teva Pharmaceut. Works Private Ltd. Co, as houseflies and stable flies. Most cases of gastric habronemiasiHungary)s , twice every second day. -
Original Papers Molecular Characterization of the First Internal
Annals of Parasitology 2015, 61(4), 241-246 Copyright© 2015 Polish Parasitological Society doi: 10.17420/ap6104.13 Original papers Molecular characterization of the first internal transcribed spacer of rDNA of Parabronema skrjabini for the first time in sheep Seyed Sajjad Hasheminasab Department of Parasitology, Faculty of Veterinary Medicine, University of Tehran, Qareeb St., Azadi Ave., 1419963111 Tehran, Iran; E-mail: [email protected] ABSTRACT. Parabronema skrjabini is a spirurid nematode of the family Habronematidae that lives in the abomasum of ruminants such as sheep and goats. The purpose of this study was to investigate the molecular aspects of Parabronema skrjabini in sheep. The worms were collected from sheep in Sanandaj (west of Iran). The first internal transcribed spacer (ITS) of ribosomal DNA (rDNA) nucleotide fragments of Parabronema skrjabini were amplified by polymerase chain reaction (PCR) using two pairs of specific primers (Para-Ir-R and Para-Ir-F). ITS1 homology in the sequence of this study was 69% compared with the sequence data in GenBank. To our knowledge, this is the first study in the world exploring the genetic diversity of P. skrjabini in sheep based on ITS1. Key words: Parabronema skrjabini , PCR, Sanandaj, Iran Introduction candidate [15]. A range of studies has demonstrated that polymerase chain reaction (PCR)-based Parabronema skrjabini is one of the nematodes approaches can be used for the species specific that occurs in the abomasum of ruminants and has a identification of parasitic nematodes (from different wide distribution in Africa, Asia and some orders), irrespective of developmental stage [16]. P. Mediterranean countries. -
(Nematoda: Habronematidae) from the Burchelps Zebras and Hartmann's Mountain Zebras in Southern Africa
Proc. Helminthol. Soc. Wash. 56(2), 1989, pp. 183-191 Habronema malani sp. n. and Habronema tomasi sp. n. (Nematoda: Habronematidae) from the BurchelPs Zebras and Hartmann's Mountain Zebras in Southern Africa ROSINA C. KRECEK Department of Parasitology, Faculty of Veterinary Science, University of Pretoria, Private Bag X04, Onderstepoort 0110, South Africa ABSTRACT: Habronema malani sp. n. is described from the stomachs of 44 Burchell's zebras, Equus burchelli antiquorum, in the Etosha and Kruger national parks and 6 Hartmann's mountain zebras, Equus zebra hart- mannae, from the Etosha National Park in southern Africa. Habronema tomasi sp. n. is described from the small intestines of 35 Burchell's zebras in the Kruger National Park. Habronema malani is distinguished from other members of the genus by its deep buccal capsule with walls that are narrower anteriorly than posteriorly and have projections in the anterior end; spicule length ratio (right:left) ranging 1:2.3 to 1:3.7; a short, stout, and striated right spicule; and a long and slender left spicule with a pointed projection. Habronema tomasi is differentiated from the other species by buccal capsule walls that are wider anteriorly than posteriorly; a distance between the anterior wall of the buccal capsule and the inner surface of the lateral lips that is almost equal to the buccal capsule depth; an ovejector with spiral-shaped muscles; and a spicule length ratio (right: left) ranging 1:1.5 to 1:2.95. The right spicule of//, tomasi is short and cross striated except at the distal fourth where the tip is flanged. -
ICAR-JRF Tutorial Question Bank. 2014. Veterinary College, KVAFSU
KARNATAKA VETERINARY, ANIMAL AND FISHERIES SCIENCES UNIVERSITY, BIDAR-585 401 ICAR-JRF [Indian Council of Agricultural Research, New Delhi] TUTORIAL QUESTION BANK FOR THE BENEFIT OF: FINAL YEAR B.V.Sc & A.H STUDENTS VETERINARY COLLEGE, VINOBANAGAR SHIMOGA-577204 Tutorial Classes Conducted under SCP- TSP Grant from the Government of Karnataka [FY: 2013-14] Organized by: VETERINARY COLLEGE, SHIMOGA Karnataka Veterinary, Animal &Fisheries Sciences University Vinobanagar, Shimoga-577204 E.mail: [email protected] Tel: 08182-651001 KARNATAKA VETERINARY, ANIMAL AND FISHERIES SCIENCES UNIVERSITY, BIDAR-585 401 ICAR-JRF 1 [Indian Council of Agricultural Research, New Delhi] TUTORIAL QUESTION BANK FOR THE BENEFIT OF: FINAL YEAR B.V.Sc & A.H STUDENTS VETERINARY COLLEGE, VINOBANAGAR SHIMOGA-577204 Prepared by: Dr. PRAKSH NADOOR Professor& Head Department of Pharmacology & Toxicology Veterinary College, Shimoga-577204 Dr. LOKESH L.V Assistant Professor Department of Pharmacology & Toxicology Veterinary College, Shimoga-577204 and Dr.NAGARAJA . L Assistant Professor Department of Veterinary Medicine Veterinary College, Shimoga-577204 2 FORE WORD The Indian Council of Council Agricultural Research [ICAR],New Delhi is the apex body for co-ordinating, guiding and managing research and education in agriculture including horticulture, fisheries and animal sciences in the entire country. With 99 ICAR institutes and 53 agricultural universities (including veterinary universities) spread across the country this is one of the largest national agricultural systems in the world. Apart from above mandates of the Council, it is also encourgining student’s to undertake quality higher education in veterinary, agriculture, horticulture, forestry and fishery sciences, in a way to produce quality scientists required for not only for its premier research institutes spread across the country, but also to scale up with human resource development. -
Stablefly Bibliography
United States Department of Agriculture A Century and a Half of Agricultural Research Research on the Stable Service ARS-173 Fly, Stomoxys calcitrans July 2012 (L.) (Diptera: Muscidae), 1862-2011: An Annotated Bibliography United States Department of A Century and a Half of Agriculture Agricultural Research on the Stable Fly, Research Service Stomoxys calcitrans (L.) ARS-173 (Diptera: Muscidae), 1862-2011: July 2012 An Annotated Bibliography K.M. Kneeland, S.R. Skoda, J.A. Hogsette, A.Y. Li, J. Molina-Ochoa, K.H. Lohmeyer, and J.E. Foster _____________________________ Kneeland, Molina-Ochoa, and Foster are with the Department of Entomology, University of Nebraska, Lincoln, NE. Molina-Ochoa also is the Head of Research and Development, Nutrilite SRL de CV, El Petacal, Jalisco, Mexico. Skoda is with the Knipling-Bushland U.S. Livestock Insects Research Laboratory (KBUSLIRL), Screwworm Research Unit, USDA Agricultural Research Service, Kerrville, TX. Hogsette is with the Center for Medical, Agricultural and Veterinary Entomology, USDA Agricultural Research Service, Gainesville, FL. Li and Lohmeyer are with KBUSLIRL, Tick and Biting Fly Research Unit, USDA Agricultural Research Service, Kerrville, TX. Abstract • sustain a competitive agricultural economy; • enhance the natural resource base and the Kneeland, K.M., S.R. Skoda, J.A. Hogsette, environment; and A.Y. Li, J. Molina-Ochoa, K.H. Lohmeyer, • provide economic opportunities for rural and J.E. Foster. 2012. A Century and a Half of citizens, communities, and society as a Research on the Stable Fly, Stomoxys whole. calcitrans (L.) (Diptera: Muscidae), 1862- 2011: An Annotated Bibliography. ARS-173. Mention of trade names or commercial U.S. -
Helminths of Domestic Ecruids
•-'"•--•' \ _*- -*."••', ~ J "~ __ . Helminths • , ('~ "V- ' '' • •'•',' •-',',/ r , : '•' '' .i X -L-' (( / ." J'f V^'-: of Domestic Ecruids £? N- .•'<••.•;• c-N-:-,'1. '.fil f^VvX , -J-J •J v-"7\c- : r ¥ : : : •3r'vNf l' J-.i' !;T'/ •:-.' ' -' >C>.'M ' -A-: ^CV •-'. ^,-• . -.V '^/,^' ^7 /v' • W;---\ '' •• x ;•- '„•-': Illustrated Keys x to Genera arid Species with Emphasis on ISForth Americain Forms •v '- ;o,/o- .-T-N. - 'vvX: >'"v v/f-.:,;., •>'s'-'^'^ v;' • „/ - i :• -. -.' -i ' '• s,'.-,-'• \. j *" '•'- ••'•' . • - •• .•(? ° •>•)• ^ "i -viL \. .s". y:.;iv>'\- /X; .f' •^: StU.- v; ^ i f :T. RALPH LiCHTENFEts (Drawmsrs by ROBERT B. EWING) < J '; "- • " •-•J- -' ' 3^y i Volume 42 ^ ;;V December-1975 .Special Issue \ PROCEEDINGS OF ''• HELMINTHOLOGIGAL \A ,) 6t WAkHlN(3TON *! tf-j Copyright © 2011, The Helminthological~--e\. •-• "">. Society of Washington THE HELMINTHOLOGICAL SOCIETY OF WASHINGTON THE SOCIETY meets once a month from October through May for the presentation and discussion of papers in any arid all branches of parasitology or related sciences. All interested persons are invited to attend. x / ; ., . ',.-.; - / ''—,•/-; Persons interested in membership in the Helminthological Society of Washington may obtain application blanks froni the Corresponding Secretary-Treasurer,'Dr. William R. Nickle, Nema- tology Laboratory, Plant Protection Institute,- ARS-USDA, Beltsville, Maryland 20705. JA year's subscription to the Proceedings is included in the annual dues ($8.00). • ;, \ / OFFICERS OF THE SOCIETY FOR 1975 President: ROBERT S, ISENSTEIN Vice President: A. MORGAN GOLDEN v>: < Corresponding Secretary-Treasurer: WILLIAM R. NICKLE -\l Assistant Corresponding Secretary-Treasurer: KENDALL G. POWERS c •Recording Secretary: j. RALPH LICHTENFELS Librarian: JUDITH M. SHAW (1962- ) Archivist: JUDITH M. SHAW (1970-\ ), U ', , .:, 'Representative to the Washington Academy of Sciences: ~ JAMES H. -
Parasitic Diseases of Equids in Iran (1931–2020): a Literature Review
Sazmand et al. Parasites Vectors (2020) 13:586 https://doi.org/10.1186/s13071-020-04472-w Parasites & Vectors REVIEW Open Access Parasitic diseases of equids in Iran (1931– 2020): a literature review Alireza Sazmand1* , Aliasghar Bahari2 , Sareh Papi1 and Domenico Otranto1,3 Abstract Parasitic infections can cause many respiratory, digestive and other diseases and contribute to some performance conditions in equids. However, knowledge on the biodiversity of parasites of equids in Iran is still limited. The present review covers all the information about parasitic diseases of horses, donkeys, mules and wild asses in Iran published as articles in Iranian and international journals, dissertations and congress papers from 1931 to July 2020. Parasites so far described in Iranian equids include species of 9 genera of the Protozoa (Trypanosoma, Giardia, Eimeria, Klossiella, Cryptosporidium, Toxoplasma, Neospora, Theileria and Babesia), 50 helminth species from the digestive system (i.e., 2 trematodes, 3 cestodes and 37 nematodes) and from other organs (i.e., Schistosoma turkestanica, Echinococcus granulosus, Dictyocaulus arnfeldi, Paraflaria multipapillosa, Setaria equina and 3 Onchocerca spp.). Furthermore, 16 species of hard ticks, 3 mite species causing mange, 2 lice species, and larvae of 4 Gastrophilus species and Hippobosca equina have been reported from equids in Iran. Archeoparasitological fndings in coprolites of equids include Fasciola hepatica, Oxyuris equi, Anoplocephala spp. and intestinal strongyles. Parasitic diseases are important issues in terms of animal welfare, economics and public health; however, parasites and parasitic diseases of equines have not received adequate attention compared with ruminants and camels in Iran. The present review highlights the knowledge gaps related to equines about the presence, species, genotypes and subtypes of Neospora hughesi, Sarcocystis spp., Trichinella spp., Cryptosporidium spp., Giardia duodenalis, Blastocystis and microsporidia. -
Incidence of Cutaneous Habronemosis in Manipuri Ponies in India T ⁎ Chirom Nishita Devia, Sonjoy Kumar Borthakura, , Gautam Patraa, N
Veterinary Parasitology: Regional Studies and Reports 17 (2019) 100295 Contents lists available at ScienceDirect Veterinary Parasitology: Regional Studies and Reports journal homepage: www.elsevier.com/locate/vprsr Original article Incidence of cutaneous habronemosis in Manipuri ponies in India T ⁎ Chirom Nishita Devia, Sonjoy Kumar Borthakura, , Gautam Patraa, N. Shyamsana Singhb, T.C. Tolenkhombab, R. Ravindranc, Subhamoy Ghosha a Department of Veterinary Parasitology, College of Veterinary Sciences and Animal Husbandry, Central Agricultural University, Selesih, Aizawl, Mizoram, India b Department of Animal Genetics and Breeding, College of Veterinary Sciences and Animal Husbandry, Central Agricultural University, Selesih, Aizawl, Mizoram, India c Department of Veterinary Pathology, College of Veterinary Sciences and Animal Husbandry, Central Agricultural University, Selesih, Aizawl, Mizoram, India ARTICLE INFO ABSTRACT Keywords: Information pertaining to parasitic fauna and parasitic diseases in Manipuri ponies in India is not available. Manipuri pony Moreover, no systematic studies have been undertaken on cutaneous habronemosis in Manipuri ponies which is Cutaneous habronemosis a common skin problem of Manipuri ponies as reported by pony owners. Keeping in the view of the importance India of parasitic infections in veterinary health coverage particularly in Manipuri ponies, the present study was planned. A survey of natural cases of cutaneous habronemosis followed by molecular confirmation of species involved and treatments were done. Out of 200 ponies examined, nine cases (4.5%) of cutaneous habronemosis was recorded. Gross examination revealed raised and ulcerated wounds with necrotic tissues covered with yellowish-tan granulation. Histopathological study revealed eosinophilic granuloma and in the center of the granuloma with necrotic debris. Remnants of the Hebronema larvae with infiltrating neutrophils surrounded by proliferating fibrous tissue with numerous eosinophils, macrophages and lymphocytes were also observed. -
Host-Parasite Interaction in Horses: Mucosal Responses to Naturally Acquired Cyathostomin Infections and Anthelmintic Treatment
University of Kentucky UKnowledge Theses and Dissertations--Veterinary Science Veterinary Science 2020 Host-Parasite Interaction in Horses: Mucosal Responses to Naturally Acquired Cyathostomin Infections and Anthelmintic Treatment Ashley Elaine Steuer University of Kentucky, [email protected] Author ORCID Identifier: https://orcid.org/0000-0003-1063-5723 Digital Object Identifier: https://doi.org/10.13023/etd.2020.160 Right click to open a feedback form in a new tab to let us know how this document benefits ou.y Recommended Citation Steuer, Ashley Elaine, "Host-Parasite Interaction in Horses: Mucosal Responses to Naturally Acquired Cyathostomin Infections and Anthelmintic Treatment" (2020). Theses and Dissertations--Veterinary Science. 47. https://uknowledge.uky.edu/gluck_etds/47 This Doctoral Dissertation is brought to you for free and open access by the Veterinary Science at UKnowledge. It has been accepted for inclusion in Theses and Dissertations--Veterinary Science by an authorized administrator of UKnowledge. For more information, please contact [email protected]. STUDENT AGREEMENT: I represent that my thesis or dissertation and abstract are my original work. Proper attribution has been given to all outside sources. I understand that I am solely responsible for obtaining any needed copyright permissions. I have obtained needed written permission statement(s) from the owner(s) of each third-party copyrighted matter to be included in my work, allowing electronic distribution (if such use is not permitted by the fair use doctrine) which will be submitted to UKnowledge as Additional File. I hereby grant to The University of Kentucky and its agents the irrevocable, non-exclusive, and royalty-free license to archive and make accessible my work in whole or in part in all forms of media, now or hereafter known. -
Genus : Habronema
Genus : Habronema Dr. R. K. Sharma Department of Veterinary Parasitology Bihar veterinary College, Patna Bihar Animal Sciences University,Patna-14 Habronema : Morphology ▪ Habronema commonly known as stomach worm found in horses. ▪ Adult has two lateral lips, dorsal and ventral lips may also be present. ▪ Buccal capsule cylindrical and chitinous. ▪ Oesophagous is divided into two parts, a short anterior muscular and long posterior glandular portion. ▪ Intestine is simple without any diverticula. ▪ The male have unequal spicules, gubernaculum present and bears 4 pairs of preanal, 1 pair adanal and 2 pairs of postanal pedunculated papillae, 3 pairs of sessile papillae also present, ▪ Male have also a spirally twisted tail. Source -Google Source- google Habronema megastoma Dr. R.K.Sharma Source -Google Source- google Habronema megastoma Dr. R.K.Sharma SourceDraschia -Google spp. Source- google Draschia megastoma Dr. R.K.Sharma Habronema : Life cycle ▪ They have an indirect life cycle, with intermediate hosts mainly- Houseflies (Musca domestica) - Habronema muscae and Draschia megastoma Stable flies (Stomoxys calcitrans) - Habronema microstoma. ▪ Adult female worms lay eggs or release L1-larvae in the stomach of the horses that are passed with the feces. ▪ These larvae are ingested by fly maggots found in the horse manure. They further develop to infective L3 larvae inside the maggots within about 2 weeks. ▪ The fly maggots complete their development to adult flies. The infective L3 larvae migrate to the mouth of the flies from where they are deposited on the final host (horses, donkeys, etc.) visited by the flies. Habronema : Life cycle….contd ▪ They are often deposited on humid parts of the host's body (eyes, nose, lips, genitalia, open wounds, etc.) to which many flies are attracted. -
Proceedings of the Helminthological Society of Washington 5(1) 1938
VOLUME 5 JANUARY, 1938 NUMBER 1 PROCEEDINGS of The Helminthological Society of Washington EDITORIAL COMMITTEE JESSE R. CHRISTIE, Editor U. S. Bureau of Plant Industry EMMETT W . PRICE U. S. Bureau of Animal Industry GILBERT F . OTTO Johns Hopkins University HENRY E . EWING U. S. Bureau . of Entomology ALLEN MCINTOSH U. S. Bureau of Animal Industry PUBLISHED BY THE HELMINTHOLOGICAL SOCIETY OF WASHINGTON Subscription $1 .00 a volume, foreign, $1 .25 PROCEEDINGS OF THE HELMINTHOLOGICAL SOCIETY OF WASHINGTON The Proceedings of the Helminthological Society of Washington is a me- dium for the publication of notes and papers in helminthology and related sub- jects . Each volume consists of 2 numbers issued in January and July . Volume 1, number 1, was issued in April, 1934. The Proceedings are intended primarily for the publication of contributions by members of the Society but papers by persons who are not members will be accepted provided the author will contribute toward the cost of publication . Manuscripts may be sent to any member of the editorial committee . Manu- scripts must be typewritten (double spaced) and submitted in finished form for transmission to the p inter. Authors should not confine themselves to merely a statement of conclusions but should present a clear indication of the methods and procedures by which the conclusions were derived. Except in the case of manu- scripts specifically designated as preliminary papers to be published in extenso later, a manuscript is accepted with the understanding that it is not to be pub- lished, with essentially the same material, elsewhere . To appear in the January number, manuscripts should be received by the editor not later than December first ; to appear in the July number, not later than June first.