Drosophila</I> T-Box Transcription Factor Midline Functions Within the Insulin/AKT and C-Jun-N-Terminal Kinas
Total Page:16
File Type:pdf, Size:1020Kb
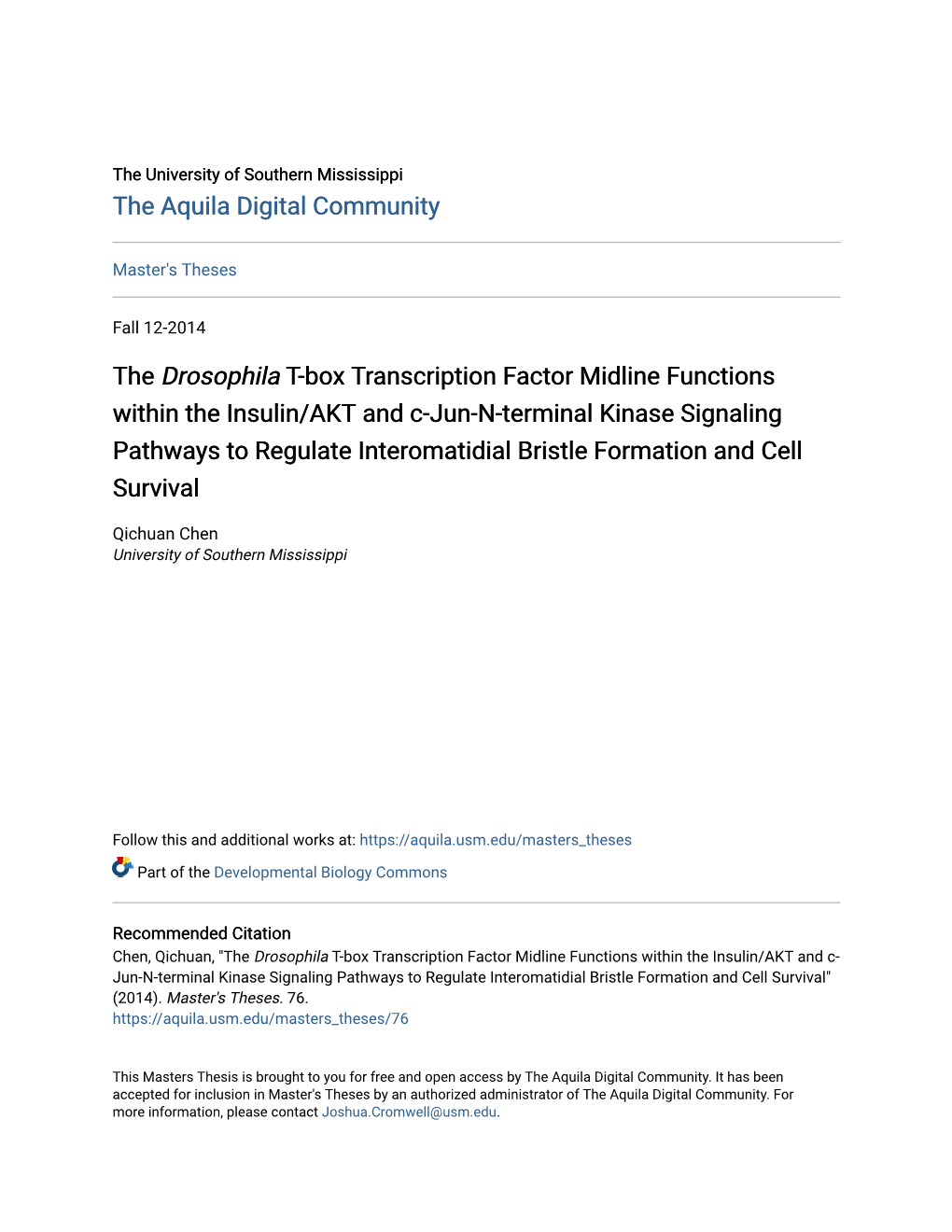
Load more
Recommended publications
-
TBX15/Mir-152/KIF2C Pathway Regulates Breast Cancer Doxorubicin Resistance Via Preventing PKM2 Ubiquitination
TBX15/miR-152/KIF2C Pathway Regulates Breast Cancer Doxorubicin Resistance via Preventing PKM2 Ubiquitination Cheng-Fei Jiang Nanjing Medical University Yun-Xia Xie Zhengzhou University Ying-Chen Qian Nanjing Medical University Min Wang Nanjing Medical University Ling-Zhi Liu Thomas Jefferson University - Center City Campus: Thomas Jefferson University Yong-Qian Shu Nanjing Medical University Xiao-Ming Bai ( [email protected] ) Nanjing Medical University Bing-Hua Jiang Thomas Jefferson University Research Keywords: TBX15, miR-152, KIF2C, PKM2, Doxorubicin resistance, breast cancer Posted Date: December 28th, 2020 DOI: https://doi.org/10.21203/rs.3.rs-130874/v1 License: This work is licensed under a Creative Commons Attribution 4.0 International License. Read Full License Page 1/29 Abstract Background Chemoresistance is a critical risk problem for breast cancer treatment. However, mechanisms by which chemoresistance arises remains to be elucidated. The expression of T-box transcription factor 15 (TBX- 15) was found downregulated in some cancer tissues. However, role and mechanism of TBX15 in breast cancer chemoresistance is unknown. Here we aimed to identify the effects and mechanisms of TBX15 in doxorubicin resistance in breast cancer. Methods As measures of Drug sensitivity analysis, MTT and IC50 assays were used in DOX-resistant breast cancer cells. ECAR and OCR assays were used to analyze the glycolysis level, while Immunoblotting and Immunouorescence assays were used to analyze the autophagy levels in vitro. By using online prediction software, luciferase reporter assays, co-Immunoprecipitation, Western blotting analysis and experimental animals models, we further elucidated the mechanisms. Results We found TBX15 expression levels were decreased in Doxorubicin (DOX)-resistant breast cancer cells. -
Table S1 the Four Gene Sets Derived from Gene Expression Profiles of Escs and Differentiated Cells
Table S1 The four gene sets derived from gene expression profiles of ESCs and differentiated cells Uniform High Uniform Low ES Up ES Down EntrezID GeneSymbol EntrezID GeneSymbol EntrezID GeneSymbol EntrezID GeneSymbol 269261 Rpl12 11354 Abpa 68239 Krt42 15132 Hbb-bh1 67891 Rpl4 11537 Cfd 26380 Esrrb 15126 Hba-x 55949 Eef1b2 11698 Ambn 73703 Dppa2 15111 Hand2 18148 Npm1 11730 Ang3 67374 Jam2 65255 Asb4 67427 Rps20 11731 Ang2 22702 Zfp42 17292 Mesp1 15481 Hspa8 11807 Apoa2 58865 Tdh 19737 Rgs5 100041686 LOC100041686 11814 Apoc3 26388 Ifi202b 225518 Prdm6 11983 Atpif1 11945 Atp4b 11614 Nr0b1 20378 Frzb 19241 Tmsb4x 12007 Azgp1 76815 Calcoco2 12767 Cxcr4 20116 Rps8 12044 Bcl2a1a 219132 D14Ertd668e 103889 Hoxb2 20103 Rps5 12047 Bcl2a1d 381411 Gm1967 17701 Msx1 14694 Gnb2l1 12049 Bcl2l10 20899 Stra8 23796 Aplnr 19941 Rpl26 12096 Bglap1 78625 1700061G19Rik 12627 Cfc1 12070 Ngfrap1 12097 Bglap2 21816 Tgm1 12622 Cer1 19989 Rpl7 12267 C3ar1 67405 Nts 21385 Tbx2 19896 Rpl10a 12279 C9 435337 EG435337 56720 Tdo2 20044 Rps14 12391 Cav3 545913 Zscan4d 16869 Lhx1 19175 Psmb6 12409 Cbr2 244448 Triml1 22253 Unc5c 22627 Ywhae 12477 Ctla4 69134 2200001I15Rik 14174 Fgf3 19951 Rpl32 12523 Cd84 66065 Hsd17b14 16542 Kdr 66152 1110020P15Rik 12524 Cd86 81879 Tcfcp2l1 15122 Hba-a1 66489 Rpl35 12640 Cga 17907 Mylpf 15414 Hoxb6 15519 Hsp90aa1 12642 Ch25h 26424 Nr5a2 210530 Leprel1 66483 Rpl36al 12655 Chi3l3 83560 Tex14 12338 Capn6 27370 Rps26 12796 Camp 17450 Morc1 20671 Sox17 66576 Uqcrh 12869 Cox8b 79455 Pdcl2 20613 Snai1 22154 Tubb5 12959 Cryba4 231821 Centa1 17897 -
Supplemental Materials ZNF281 Enhances Cardiac Reprogramming
Supplemental Materials ZNF281 enhances cardiac reprogramming by modulating cardiac and inflammatory gene expression Huanyu Zhou, Maria Gabriela Morales, Hisayuki Hashimoto, Matthew E. Dickson, Kunhua Song, Wenduo Ye, Min S. Kim, Hanspeter Niederstrasser, Zhaoning Wang, Beibei Chen, Bruce A. Posner, Rhonda Bassel-Duby and Eric N. Olson Supplemental Table 1; related to Figure 1. Supplemental Table 2; related to Figure 1. Supplemental Table 3; related to the “quantitative mRNA measurement” in Materials and Methods section. Supplemental Table 4; related to the “ChIP-seq, gene ontology and pathway analysis” and “RNA-seq” and gene ontology analysis” in Materials and Methods section. Supplemental Figure S1; related to Figure 1. Supplemental Figure S2; related to Figure 2. Supplemental Figure S3; related to Figure 3. Supplemental Figure S4; related to Figure 4. Supplemental Figure S5; related to Figure 6. Supplemental Table S1. Genes included in human retroviral ORF cDNA library. Gene Gene Gene Gene Gene Gene Gene Gene Symbol Symbol Symbol Symbol Symbol Symbol Symbol Symbol AATF BMP8A CEBPE CTNNB1 ESR2 GDF3 HOXA5 IL17D ADIPOQ BRPF1 CEBPG CUX1 ESRRA GDF6 HOXA6 IL17F ADNP BRPF3 CERS1 CX3CL1 ETS1 GIN1 HOXA7 IL18 AEBP1 BUD31 CERS2 CXCL10 ETS2 GLIS3 HOXB1 IL19 AFF4 C17ORF77 CERS4 CXCL11 ETV3 GMEB1 HOXB13 IL1A AHR C1QTNF4 CFL2 CXCL12 ETV7 GPBP1 HOXB5 IL1B AIMP1 C21ORF66 CHIA CXCL13 FAM3B GPER HOXB6 IL1F3 ALS2CR8 CBFA2T2 CIR1 CXCL14 FAM3D GPI HOXB7 IL1F5 ALX1 CBFA2T3 CITED1 CXCL16 FASLG GREM1 HOXB9 IL1F6 ARGFX CBFB CITED2 CXCL3 FBLN1 GREM2 HOXC4 IL1F7 -
Tbx15 Defines a Glycolytic Subpopulation and White Adipocyte
2822 Diabetes Volume 66, November 2017 Tbx15 Defines a Glycolytic Subpopulation and White Adipocyte Heterogeneity Kevin Y. Lee,1,2,3 Rita Sharma,2,3 Grant Gase,2,3 Siegfried Ussar,1,4,5 Yichao Li,6 Lonnie Welch,6 Darlene E. Berryman,2,3 Andreas Kispert,7 Matthias Bluher,8 and C. Ronald Kahn1 Diabetes 2017;66:2822–2829 | https://doi.org/10.2337/db17-0218 Tbx15 is a member of the T-box gene family of mesoder- adipose tissue (WAT) is associated with insulin resistance and mal developmental genes. We have recently shown that increased risk of metabolic disease, whereas subcutaneous WAT Tbx15 plays a critical role in the formation and metabolic maybeprotectiveagainstthedevelopmentofmetabolic programming of glycolytic myofibers in skeletal muscle. disorders (1). We and others (2,3) have shown that differ- Tbx15 is also differentially expressed among white adi- ences in WAT depots are also marked by differential expres- pose tissue (WAT) in different body depots. In the current sion of developmental genes. study, using three independent methods, we show that Tbx15 is a member of the T-box gene family of develop- even within a single WAT depot, high Tbx15 expression mental genes and has an established role in skeletal forma- is restricted to a subset of preadipocytes and mature fi tion (4). We have recently shown that in skeletal muscle, white adipocytes. Gene expression and metabolic pro l- fi ing demonstrate that the Tbx15Hi preadipocyte and adipo- Tbx15 is highly and speci cally expressed in glycolytic fi fi cyte subpopulations of cells are highly glycolytic, whereas myo bers and regulates the metabolism of these myo bers Tbx15Low preadipocytes and adipocytes in the same de- (5). -
1714 Gene Comprehensive Cancer Panel Enriched for Clinically Actionable Genes with Additional Biologically Relevant Genes 400-500X Average Coverage on Tumor
xO GENE PANEL 1714 gene comprehensive cancer panel enriched for clinically actionable genes with additional biologically relevant genes 400-500x average coverage on tumor Genes A-C Genes D-F Genes G-I Genes J-L AATK ATAD2B BTG1 CDH7 CREM DACH1 EPHA1 FES G6PC3 HGF IL18RAP JADE1 LMO1 ABCA1 ATF1 BTG2 CDK1 CRHR1 DACH2 EPHA2 FEV G6PD HIF1A IL1R1 JAK1 LMO2 ABCB1 ATM BTG3 CDK10 CRK DAXX EPHA3 FGF1 GAB1 HIF1AN IL1R2 JAK2 LMO7 ABCB11 ATR BTK CDK11A CRKL DBH EPHA4 FGF10 GAB2 HIST1H1E IL1RAP JAK3 LMTK2 ABCB4 ATRX BTRC CDK11B CRLF2 DCC EPHA5 FGF11 GABPA HIST1H3B IL20RA JARID2 LMTK3 ABCC1 AURKA BUB1 CDK12 CRTC1 DCUN1D1 EPHA6 FGF12 GALNT12 HIST1H4E IL20RB JAZF1 LPHN2 ABCC2 AURKB BUB1B CDK13 CRTC2 DCUN1D2 EPHA7 FGF13 GATA1 HLA-A IL21R JMJD1C LPHN3 ABCG1 AURKC BUB3 CDK14 CRTC3 DDB2 EPHA8 FGF14 GATA2 HLA-B IL22RA1 JMJD4 LPP ABCG2 AXIN1 C11orf30 CDK15 CSF1 DDIT3 EPHB1 FGF16 GATA3 HLF IL22RA2 JMJD6 LRP1B ABI1 AXIN2 CACNA1C CDK16 CSF1R DDR1 EPHB2 FGF17 GATA5 HLTF IL23R JMJD7 LRP5 ABL1 AXL CACNA1S CDK17 CSF2RA DDR2 EPHB3 FGF18 GATA6 HMGA1 IL2RA JMJD8 LRP6 ABL2 B2M CACNB2 CDK18 CSF2RB DDX3X EPHB4 FGF19 GDNF HMGA2 IL2RB JUN LRRK2 ACE BABAM1 CADM2 CDK19 CSF3R DDX5 EPHB6 FGF2 GFI1 HMGCR IL2RG JUNB LSM1 ACSL6 BACH1 CALR CDK2 CSK DDX6 EPOR FGF20 GFI1B HNF1A IL3 JUND LTK ACTA2 BACH2 CAMTA1 CDK20 CSNK1D DEK ERBB2 FGF21 GFRA4 HNF1B IL3RA JUP LYL1 ACTC1 BAG4 CAPRIN2 CDK3 CSNK1E DHFR ERBB3 FGF22 GGCX HNRNPA3 IL4R KAT2A LYN ACVR1 BAI3 CARD10 CDK4 CTCF DHH ERBB4 FGF23 GHR HOXA10 IL5RA KAT2B LZTR1 ACVR1B BAP1 CARD11 CDK5 CTCFL DIAPH1 ERCC1 FGF3 GID4 HOXA11 IL6R KAT5 ACVR2A -
Tbx22 Expressions During Palatal Development in Fetuses with Glucocorticoid-/ Alcohol-Induced C57BL/6N Cleft Palates
ORIGINAL ARTICLE Tbx22 Expressions During Palatal Development in Fetuses With Glucocorticoid-/ Alcohol-Induced C57BL/6N Cleft Palates Soung Min Kim, DDS, MSD, PhD,* Jong Ho Lee, DDS, MSD, PhD,* Samir Jabaiti, MD, FRCS(Edin),Þ Suk Keun Lee, DDS, MSD, PhD,þ and Jin Young Choi, DDS, MD, PhD* between the palatal shelves, where 2 palatal shelves had fused as in Abstract: T-box transcription factor 22 (Tbx22) belongs to the T-box normal development but failed to meet and fuse to each other. By in family of transcription factors and was originally found using an in situ hybridization, Tbx22 mRNAwas found to be expressed in distinct silico approach to identify new genes in the human Xq12-Xq21 re- areas of the head, such as the mesenchyme of the inferior nasal septum, gion. Mutations in Tbx22 have been reported in families with X-linked the posterior palatal shelf before fusion, and the attachment of the cleft palate and ankyloglossia, but the underlying pathogenetic mech- tongue during normal development of the palate and maxilla from anism remains unknown. The aim of this study was to evaluate the GD 11.5. Localization in the tongue frenum correlated with the expression of Tbx22 messenger RNA (mRNA) during palatogenesis ankyloglossia phenotype in the induced cleft palate animal model. in glucocorticoid-/alcohol-induced cleft palate in a C57BL/6N mouse model. Palatal development was monitored by histomorphologic and Key Words: Alcohol, ankyloglossia, cleft palate, C57BL/6N immunohistochemical studies and by in situ hybridization. Thirty mouse, glucocorticoid, nasal septum, T-box transcription factor, pregnant C57BL/6N mice at 8 weeks of age, weighing 20 to 25 g, were Tbx22 used in this study. -
Supplementary Table 1
Supplementary Table 1. 492 genes are unique to 0 h post-heat timepoint. The name, p-value, fold change, location and family of each gene are indicated. Genes were filtered for an absolute value log2 ration 1.5 and a significance value of p ≤ 0.05. Symbol p-value Log Gene Name Location Family Ratio ABCA13 1.87E-02 3.292 ATP-binding cassette, sub-family unknown transporter A (ABC1), member 13 ABCB1 1.93E-02 −1.819 ATP-binding cassette, sub-family Plasma transporter B (MDR/TAP), member 1 Membrane ABCC3 2.83E-02 2.016 ATP-binding cassette, sub-family Plasma transporter C (CFTR/MRP), member 3 Membrane ABHD6 7.79E-03 −2.717 abhydrolase domain containing 6 Cytoplasm enzyme ACAT1 4.10E-02 3.009 acetyl-CoA acetyltransferase 1 Cytoplasm enzyme ACBD4 2.66E-03 1.722 acyl-CoA binding domain unknown other containing 4 ACSL5 1.86E-02 −2.876 acyl-CoA synthetase long-chain Cytoplasm enzyme family member 5 ADAM23 3.33E-02 −3.008 ADAM metallopeptidase domain Plasma peptidase 23 Membrane ADAM29 5.58E-03 3.463 ADAM metallopeptidase domain Plasma peptidase 29 Membrane ADAMTS17 2.67E-04 3.051 ADAM metallopeptidase with Extracellular other thrombospondin type 1 motif, 17 Space ADCYAP1R1 1.20E-02 1.848 adenylate cyclase activating Plasma G-protein polypeptide 1 (pituitary) receptor Membrane coupled type I receptor ADH6 (includes 4.02E-02 −1.845 alcohol dehydrogenase 6 (class Cytoplasm enzyme EG:130) V) AHSA2 1.54E-04 −1.6 AHA1, activator of heat shock unknown other 90kDa protein ATPase homolog 2 (yeast) AK5 3.32E-02 1.658 adenylate kinase 5 Cytoplasm kinase AK7 -
2014 ADA Posters 1319-2206.Indd
INTEGRATED PHYSIOLOGY—INSULINCATEGORY SECRETION IN VIVO 1738-P increase in tumor size and pulmonary metastasis is observed, compared Sustained Action of Ceramide on Insulin Signaling in Muscle Cells: to wild type mice. In this study, we aimed to determine the mechanisms Implication of the Double-Stranded RNA Activated Protein Kinase through which hyperinsulinemia and the canonical IR signaling pathway drive RIMA HAGE HASSAN, ISABELLE HAINAULT, AGNIESZKA BLACHNIO-ZABIELSKA, tumor growth and metastasis. 100,000 MVT-1 (c-myc/vegf overexpressing) RANA MAHFOUZ, OLIVIER BOURRON, PASCAL FERRÉ, FABIENNE FOUFELLE, ERIC cells were injected orthotopically into 8-10 week old MKR mice. MKR mice HAJDUCH, Paris, France, Białystok, Poland developed signifi cantly larger MVT-1 (353.29±44mm3) tumor volumes than Intramyocellular accumulation of fatty acid derivatives like ceramide plays control mice (183.21±47mm3), p<0.05 with more numerous pulmonary a crucial role in altering the insulin message. If short-term action of ceramide metastases. Western blot and immunofl uorescent staining of primary tumors inhibits the protein kinase B (PKB/Akt), long-term action of ceramide on insulin showed an increase in vimentin, an intermediate fi lament, typically expressed signaling is less documented. Short-term treatment of either the C2C12 cell in cells of mesenchymal origin, and c-myc, a known transcription factor. Both line or human myotubes with palmitate (ceramide precursor, 16h) or directly vimentin and c-myc are associated with cancer metastasis. To assess if insulin with ceramide (2h) induces a loss of the insulin signal through the inhibition and IR signaling directly affects the expression these markers, in vitro studies of PKB/Akt. -
Genetic Factors in Nonsyndromic Orofacial Clefts
Published online: 2021-02-12 THIEME Review Article 101 Genetic Factors in Nonsyndromic Orofacial Clefts Mahamad Irfanulla Khan1 Prashanth C.S.2 Narasimha Murthy Srinath3 1 Department of Orthodontics & Dentofacial Orthopedics, The Oxford Address for correspondence Mahamad Irfanulla Khan, BDS, MDS, Dental College, Bangalore, Karnataka, India Department of Orthodontics & Dentofacial Orthopedics, The Oxford 2 Department of Orthodontics & Dentofacial Orthopedics, DAPM R.V. Dental College, Bangalore, Karnataka 560068, India Dental College, Bangalore, Karnataka, India (e-mail: [email protected]). 3 Department of Oral & Maxillofacial Surgery, Krishnadevaraya College of Dental Sciences, Bangalore, Karnataka, India Global Med Genet 2020;7:101–108. Abstract Orofacial clefts (OFCs) are the most common congenital birth defects in humans and immediately recognized at birth. The etiology remains complex and poorly understood and seems to result from multiple genetic and environmental factors along with gene– environment interactions. It can be classified into syndromic (30%) and nonsyndromic (70%) clefts. Nonsyndromic OFCs include clefts without any additional physical or Keywords cognitive deficits. Recently, various genetic approaches, such as genome-wide associ- ► orofacial clefts ation studies (GWAS), candidate gene association studies, and linkage analysis, have ► nonsyndromic identified multiple genes involved in the etiology of OFCs. ► genetics This article provides an insight into the multiple genes involved in the etiology of OFCs. ► gene mutation Identification of specific genetic causes of clefts helps in a better understanding of the ► genome-wide molecular pathogenesis of OFC. In the near future, it helps to provide a more accurate association study diagnosis, genetic counseling, personalized medicine for better clinical care, and ► linkage analysis prevention of OFCs. -
Genetics of Lipedema: New Perspectives on Genetic Research and Molecular Diagnoses S
European Review for Medical and Pharmacological Sciences 2019; 23: 5581-5594 Genetics of lipedema: new perspectives on genetic research and molecular diagnoses S. PAOLACCI1, V. PRECONE2, F. ACQUAVIVA3, P. CHIURAZZI4,5, E. FULCHERI6,7, M. PINELLI3,8, F. BUFFELLI9,10, S. MICHELINI11, K.L. HERBST12, V. UNFER13, M. BERTELLI2; GENEOB PROJECT 1MAGI’S LAB, Rovereto (TN), Italy 2MAGI EUREGIO, Bolzano, Italy 3Department of Translational Medicine, Section of Pediatrics, Federico II University, Naples, Italy 4Istituto di Medicina Genomica, Fondazione A. Gemelli, Università Cattolica del Sacro Cuore, Rome, Italy 5UOC Genetica Medica, Fondazione Policlinico Universitario “A. Gemelli” IRCCS, Rome, Italy 6Fetal and Perinatal Pathology Unit, IRCCS Istituto Giannina Gaslini, Genoa, Italy 7Department of Integrated Surgical and Diagnostic Sciences, University of Genoa, Genoa, Italy 8Telethon Institute of Genetics and Medicine (TIGEM), Pozzuoli, Italy 9Fetal and Perinatal Pathology Unit, IRCCS Istituto Giannina Gaslini, Genoa, Italy 10Department of Neuroscience, Rehabilitation, Ophthalmology, Genetics and Maternal-Infantile Sciences, University of Genoa, Genoa, Italy 11Department of Vascular Rehabilitation, San Giovanni Battista Hospital, Rome, Italy 12Department of Medicine, University of Arizona, Tucson, AZ, USA 13Department of Developmental and Social Psychology, Faculty of Medicine and Psychology, Sapienza University of Rome, Rome, Italy Abstract. – OBJECTIVE: The aim of this quali- Introduction tative review is to provide an update on the cur- rent understanding of the genetic determinants of lipedema and to develop a genetic test to dif- Lipedema is an underdiagnosed chronic debil- ferentiate lipedema from other diagnoses. itating disease characterized by bruising and pain MATERIALS AND METHODS: An electronic and excess of subcutaneous adipose tissue of the search was conducted in MEDLINE, PubMed, and legs and/or arms in women during or after times Scopus for articles published in English up to of hormone change, especially in puberty1. -
Molecular Anatomy of Palate Development
RESEARCH ARTICLE Molecular Anatomy of Palate Development Andrew S. Potter, S. Steven Potter* Cincinnati Children’s Medical Center, Division of Developmental Biology, 3333 Burnet Ave., Cincinnati, OH, 45229, United States of America * [email protected] Abstract The NIH FACEBASE consortium was established in part to create a central resource for craniofacial researchers. One purpose is to provide a molecular anatomy of craniofacial development. To this end we have used a combination of laser capture microdissection and RNA-Seq to define the gene expression programs driving development of the murine pal- ate. We focused on the E14.5 palate, soon after medial fusion of the two palatal shelves. The palate was divided into multiple compartments, including both medial and lateral, as well as oral and nasal, for both the anterior and posterior domains. A total of 25 RNA-Seq datasets were generated. The results provide a comprehensive view of the region specific expression of all transcription factors, growth factors and receptors. Paracrine interactions can be inferred from flanking compartment growth factor/receptor expression patterns. The results are validated primarily through very high concordance with extensive previously OPEN ACCESS published gene expression data for the developing palate. In addition selected immunostain Citation: Potter AS, Potter SS (2015) Molecular validations were carried out. In conclusion, this report provides an RNA-Seq based atlas of Anatomy of Palate Development. PLoS ONE 10(7): gene expression patterns driving palate development at microanatomic resolution. This e0132662. doi:10.1371/journal.pone.0132662 FACEBASE resource is designed to promote discovery by the craniofacial research Editor: Peter Hohenstein, The Roslin Institute, community. -
Identification of Shared and Unique Gene Families Associated with Oral
International Journal of Oral Science (2017) 9, 104–109 OPEN www.nature.com/ijos ORIGINAL ARTICLE Identification of shared and unique gene families associated with oral clefts Noriko Funato and Masataka Nakamura Oral clefts, the most frequent congenital birth defects in humans, are multifactorial disorders caused by genetic and environmental factors. Epidemiological studies point to different etiologies underlying the oral cleft phenotypes, cleft lip (CL), CL and/or palate (CL/P) and cleft palate (CP). More than 350 genes have syndromic and/or nonsyndromic oral cleft associations in humans. Although genes related to genetic disorders associated with oral cleft phenotypes are known, a gap between detecting these associations and interpretation of their biological importance has remained. Here, using a gene ontology analysis approach, we grouped these candidate genes on the basis of different functional categories to gain insight into the genetic etiology of oral clefts. We identified different genetic profiles and found correlations between the functions of gene products and oral cleft phenotypes. Our results indicate inherent differences in the genetic etiologies that underlie oral cleft phenotypes and support epidemiological evidence that genes associated with CL/P are both developmentally and genetically different from CP only, incomplete CP, and submucous CP. The epidemiological differences among cleft phenotypes may reflect differences in the underlying genetic causes. Understanding the different causative etiologies of oral clefts is