Chapter Three Ammonia and the Environment
Total Page:16
File Type:pdf, Size:1020Kb
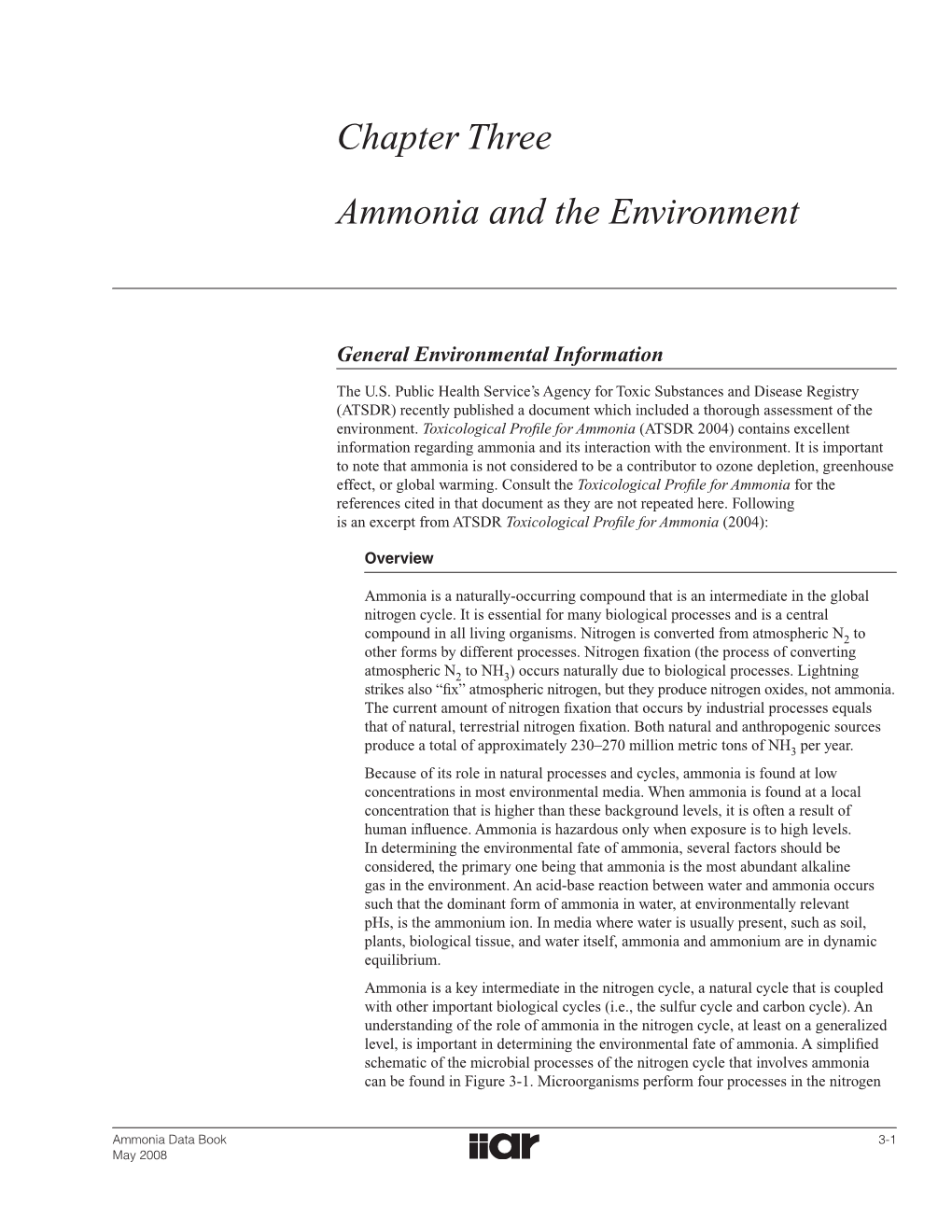
Load more
Recommended publications
-
Nitrogen Transformation Stages Into Ammonia in Broiler Production: Sources, Deposition, Transformation, and Emission Into the • Environment
Nitrogen transformation stages into ammonia in broiler production: sources, deposition, transformation, and emission into the • environment Monique de Oliveira Vilela a, Richard S. Gates b, Cecília F. Souza a, Carlos G. S. Teles Junior a a & Fernanda C. Sousa a Department of Agricultural Engineering of Federal University of Viçosa, Viçosa, Brazil, [email protected], [email protected], [email protected], [email protected] b Department of Agricultural and Biosystems Engineering, and Animal Science, Egg Industry Center, Iowa State University, Ames, USA. [email protected] Received: November 2nd, 2019. Received in revised version: July 3rd, 2020. Accepted: July 16th, 2020. Abstract Air quality is a major factor in intensive livestock management because air is the main vehicle for the dissemination of physical, chemical, and biological agents that affect the health and welfare of animals and humans. Ammonia is the main gaseous pollutant generated in poultry production. In this study, a literature review was conducted to highlight each nitrogen transformation stage during poultry production, while explaining the conversion mechanisms from the ingestion of crude protein to nitrogen volatilization into the atmosphere as ammonia gas. In summary, the steps for the synthesis of uric acid and the excretion of poultry, mineralization of nitrogen present in excreta, and volatilization of ammonia from litter are presented. Based on this review, the importance of understanding the sources and processes involved in ammonia generation and emission in poultry production is clear, thereby allowing the adoption of assertive measures that could minimize negative effects caused by ammonia emission. Keywords: air pollution; air quality; nitrate; nitrite; poultry farming. -
Options for Treatment of Ammonia in Landfill Leachate
Options for Treatment of Ammonia in Landfill Leachate by Doctor Segweni This thesis is submitted in partial fulfilment of a Master in Water Resource Management at the Waterways Centre for Freshwater Management University of Canterbury New Zealand February 2017 Abstract Treatment of landfill leachate is often needed to remove ammonia nitrogen (free ammonia and dissolved organic nitrogen) because high concentrations are known to negatively impact on waterways and the wastewater treatment process. The objective of this study was to examine ways to reduce ammonia nitrogen in landfill leachate. The methods explored were coagulation– flocculation, adsorption and system integration methods. For coagulation–flocculation treatment: jar test experiments explored the best coagulant, effective dose, pH control, mixing regimes and the use of polyelectrolytes. Three conventional coagulants – anhydrous ferric chloride, hexahydrate ferric chloride and aluminium sulfate – were examined, alongside three commercial cationic polyelectrolytes. The best coagulant was anhydrous ferric chloride, and the coagulant dosage and pH were found to be very crucial. Anhydrous ferric chloride showed removal of about 20%, 29% and 77% of ammonia nitrogen, Chemical Oxygen Demand (COD) and colour respectively at an optimum dose of 2,000 mg/L at pH 7. The mixing regimes and polyelectrolyte additions were insignificant in ammonia nitrogen removal. Sorption using local soils (type A, B, C and D) and zeolite was also studied. The four local soils were equally ineffective in removing ammonium from landfill leachate (< 5.0% removal); in contrast, zeolite was somewhat effective (23%). Two system integrations were analysed: one between coagulation–flocculation and biological nitrification, and the other between adsorption and coagulation–flocculation. -
DG Environment) Collection and Analysis of Data for the Control of Emissions from the Spreading of Manure
European Commission (DG Environment) Collection and Analysis of Data for the Control of Emissions from the Spreading of Manure Final Report 6 January 2014 AMEC Environment & Infrastructure UK Limited in partnership with BIO Intelligence Service Copyright and Non-Disclosure Notice The contents and layout of this report are subject to copyright owned by AMEC (©AMEC Environment & Infrastructure UK Limited 2013). save to the extent that copyright has been legally assigned by us to another party or is used by AMEC under licence. To the extent that we own the copyright in this report, it may not be copied or used without our prior written agreement for any purpose other than the purpose indicated in this report. The methodology (if any) contained in this report is provided to you in confidence and must not be disclosed or copied to third parties without the prior written agreement of AMEC. Disclosure of that information may constitute an actionable breach of confidence or may otherwise prejudice our commercial interests. Any third party who obtains access to this report by any means will, in any event, be subject to the Third Party Disclaimer set out below. Third-Party Disclaimer Any disclosure of this report to a third party is subject to this disclaimer. The report was prepared by AMEC at the instruction of, and for use by, our client named on the front of the report. It does not in any way constitute advice to any third party who is able to access it by any means. AMEC excludes to the fullest extent lawfully permitted all liability whatsoever for any loss or damage howsoever arising from reliance on the contents of this report. -
Ammoniacal Nitrogen and COD Removal from Stabilized Landfill
Desalination and Water Treatment 192 (2020) 111–117 www.deswater.com July doi: 10.5004/dwt.2020.25470 Ammoniacal nitrogen and COD removal from stabilized landfill leachate using granular activated carbon and green mussel (Perna viridis) shell powder as a composite adsorbent Zawawi Dauda,*, Amir Dethoa,b, Mohd Arif Roslic, Mahmoud Hijab Abubakard, Kamran Ahmed Samoe, Nur Faizan Mohammad Raisa, Azhar Abdul Halimf, Husnul Azan Tajaruding aFaculty of Civil and Environmental Engineering, Universiti Tun Hussein Onn Malaysia, emails: [email protected] (Z. Daud), [email protected] (A. Detho), [email protected] (N.F. Mohammad Rais) bEnergy and Environment Engineering Department, Quaid-e-Awam University Engineering, Science and Technology Nawabshah, Sindh, Pakistan cFaculty of Engineering Technology, Universiti Tun Hussein Onn Malaysia, email: [email protected] (M.A. Rosli) dDepartment of Civil Engineering, Modibbo Adama University of Technology Yola, Adamawa State, Nigeria, email: [email protected] (M.H. Abubakar) eElectrical Engineering Department, Quaid-e-Awam University College of Engineering, Science & Technology Larkana, Pakistan, email: [email protected] (K.A. Samo) fSchool of Environmental and Natural Resources Sciences, Universiti Kebangsaan Malaysia, email: [email protected] (A.A. Halim) gSchool of Industrial Technologi, Universiti Sains Malaysia, email: [email protected] (H.A. Tajarudin) Received 13 September 2019; Accepted 9 January 2020 abstract Leachate is a liquid that is produced when water percolates through solid waste and contain dis- solved or suspended material from various dissolved materials and bio-decomposition process. This study describes the finding of ammoniacal nitrogen and chemical oxygen demand (COD) removal partially replacing the amount of activated carbon. -
Home Landscaping Guide for Lake Tahoe and Vicinity
Cooperative Extension Bringing the University to You Home Landscaping Guide Western Area Offices Incline Village/Washoe County John Cobourn, Water Resource Specialist 865 Tahoe Blvd., Suite 110 for P.O. Box 8208 Incline Village, NV 89452-8208 Phone: (775) 832-4144 Email: [email protected] Lake Tahoe and Vicinity Reno/Washoe County Home Landscaping Guide for Lake Tahoe and Vicinity and Tahoe Lake for Guide Landscaping Home Kerrie Badertscher, Horticulturist Sue Donaldson, Water Quality Specialist 5305 Mill St. P.O. Box 11130 Reno, NV 89520-0027 Home Landscaping Guide for Lake Tahoe and Vicinity Phone: (775) 784-4848 SPONSORED BY: Douglas County Steve Lewis, Extension Educator Backyard Conservation Program of: Ed Smith, Natural Resource Specialist Nevada Tahoe Conservation District 1329 Waterloo Lane Tahoe Resource Conservation District Gardnerville, NV 89410 USDA Natural Resources Conservation Service P.O. Box 338 Minden, NV 89423-0338 California Regional Water Quality Control Board, Lahontan Region Phone: (775) 782-9960 California Tahoe Conservancy Carson City/Storey County JoAnne Skelly, Extension Educator Incline Village General Improvement District, Waste Not 2621 Northgate Lane, Suite 15 Carson City, NV 89706-1619 Lake Tahoe Environmental Education Coalition Phone: (775) 887-2252 .Nevada Division of Environmental Protection Nevada Division of State Lands, Lake Tahoe License Plate Program Tahoe Regional Planning Agency University of California Cooperative Extention University of Nevada Cooperative Extension Mission of Cooperative Extension To discover, develop, disseminate, preserve and Cooperative Extension use knowledge to strengthen the social, eco- Bringing the University to You nomic and environmental well-being of people. Educational Bulletin–06–01 (Replaces EB–02–01 & EB–00–01) $5.00 Who to Call for Help Acknowledgments If you live in California: California Department of Forestry and Fire Protection ............(530) 541-1989 www.fire.ca.gov The authors wish to thank all the people and organizations who helped make this book a reality. -
— Table of Contents — Products
— TABLE OF CONTENTS — SPECIMEN MSDS PRODUCTS LABEL (Material safety data sheets) AZATIN® XL Biological Insecticide . .2 . .7 COMPASS™O 50 WDG Fungicide . .9 . .14 CYCOCEL® Plant Growth Regulant . .17 . .21 DECATHLON™ 20 WP Greenhouse and Nursery Insecticide . .23 . .25 INSECTICIDAL SOAP 49.52 CF Insecticide . .27 . .30 MARATHON® 1% GRANULAR Greenhouse and Nursery Insecticide . .32 . .35 MARATHON® 60 WP Greenhouse and Nursery Insecticide in Water Soluble Packaging . .38 . .42 MARATHON® II Greenhouse and Nursery Insecticide in Water Soluble Packaging . .45 . .49 PYLON® Miticide . .53 . .56 STRIKE® 50 WDG Greenhouse and Nursery Systemic Fungicide . .58 . .62 SUFFUSION™ GRANULAR Growing Media Wetting Agent . .66 . .68 SUFFUSION™ LIQUID Growing Media Wetting Agent . .69 . .71 TRIACT® 70 Broad Spectrum Fungicide / Insecticide / Miticide . .72 . .75 TRIATHLON™ Greenhouse Disinfectant . .77 . .79 CHEMIGATION BULLETIN PAGE NO. AZATIN® XL . .80 GENERAL INFORMATION DOT SHIPPING INFORMATION . .82 WORKER PROTECTION QUICK REFERENCE CHART . .83 FUNGICIDE USAGE CHART . .84 INSECTICIDE USAGE CHART . .85 MARATHON 60 WP IN WSP IRRIGATION AND HOSE END APPLICATIONS . .86 PESTICIDE DILUTIONS . .87 WEIGHTS AND MEASURES CHARTS . .88 SCOUT STICKY TRAP™ . .89 Product labels in this guide are for reference purposes only. Please refer to specific labels found on product containers prior to actual use. Always read and follow label directions carefully. The specimen labels and information contained are correct as of the publication date but are subject to change without -
Study of Ammoniacal Nitrogen Removal from Leachate of Sanitary Landfills in Hilly Terrain
International Research Journal of Engineering and Technology (IRJET) e-ISSN: 2395-0056 Volume: 06 Issue: 09 | Sep 2019 www.irjet.net p-ISSN: 2395-0072 Study of Ammoniacal Nitrogen Removal from Leachate of Sanitary Landfills in Hilly Terrain Atul Sharma1, Dr. Surjit Singh Katoch2 1M.Tech. Student NIT Hamirpur (H.P.) INDIA 2Associate Professor, CEEE, NIT Hamirpur (H.P.) INDIA ---------------------------------------------------------------------***--------------------------------------------------------------------- Abstract – Nearness of ammoniacal nitrogen in the awareness and community sensitization. Municipal solid leachate is one of the serious issue looked by administrators of waste contains a big portion of biodegradable waste that landfill. Generally, quantity of organic waste present at landfill creates smell and other problems if not handled properly. site is high; the slow leaching of this organic waste produces Most of this organic waste comes from house, restaurants, nitrogen in high concentration also there is no significant pulp and paper industries, which if not collected separately mechanism for the alteration of ammoniacal nitrogen. This end up in solid waste along with the other non- cause very high amount of ammoniacal nitrogen decomposable waste leading to larger quantity to be handled concentration in landfill leachate over a long period. A at treatment and dumping site. Also segregation of this literature review regards this shows that, ammoniacal decomposable waste at processing site is almost impossible nitrogen removal from landfill leachate is not well defined and or the efficiency remains very low. With proper management to date, especially in the adsorption treatment. In addition, planning and peoples cooperation waste segregation at there are limited studies in hilly terrain of India on this source could help by reducing burden on treatment facilities regards. -
How to Comply with Your Landspreading Permit TGN EPR 8.01
How to comply with your landspreading permit TGN EPR 8.01 Version 5 February 2017 How to comply with your landspreading permit 1 Version 5 February 2017 Document Owner: National Services/ Knowledge, Strategy & Planning Version History: Document Date Summary of Changes Version Published 1.0 2.0 3.0 February 2014 4.0 October 2014 Rebrand to NRW 5.0 February 2017 Improve formatting Amendment of references of Environmental Permitting (England and Wales) Regulations 2010 to Environmental Permitting (England and Wales) Regulations 2016 Amendment of references of Nitrate Pollution Prevention Regulations 2008 to The Nitrate Pollution Prevention (Wales) Regulations 2013 (NVZ rules) Amendment of references Code of Good Agricultural Practice/CoGAP to Code of Good Agricultural Practice for the Protection of Water, Soil and Air for Wales (2011 No.20) Amendment of references of Forestry Commission to Natural Resources Wales Amendment of references of WM2 to WM3 Published by: Natural Resources Wales Cambria House 29 Newport Road Cardiff CF24 0TP 0300 065 3000 (Mon-Fri, 8am - 6pm) [email protected] www.naturalresourceswales.gov.uk © Natural Resources Wales All rights reserved. This document may be reproduced with prior permission of Natural Resources Wales How to comply with your landspreading permit 2 Version 5 February 2017 Contents Introduction .................................................................................................................................... 7 Operations and facilities covered by this guidance -
Nitrogen Management
NITROGEN Nitrogen is the basic building block of all living • Chlorophyll: Nitrogen is a constituent of the matter, both plant and animal. Plants and animals chlorophyll molecule, the green pigment that need plentiful supplies of nitrogen to insure allows plants to use sunlight energy to respiration, growth and reproduction. convert carbon dioxide and water into food (carbohydrates). Nitrogen in its pure state is a colorless, odorless, inert gas comprising 78% of the air we breathe. Nitrogen fertilization normally increases the yield In this form it cannot be used by non-legume of grain, fruit, or forage of non-legume plants. An plants. Nitrogen must be converted by soil important effect of N is that it promotes microorganisms or industrial processing into vegetative growth. forms crops can use. Applied in correct amounts, N produces a dark There is about 35,000 tons of nitrogen over every green, healthy plant that reaches maturity more acre of land. This inexhaustible supply remains rapidly than N-deficient plants. Nitrogen can constant, because nitrogen is being returned to occasionally delay crop maturity, if applied in the atmosphere at about the same rate as it is amounts greater than the crop needs. For being removed. example, excessive rates of N on tomatoes or melons may produce large, readily growing Functions of N in Plants plants that set less fruit. Too much nitrogen may result in low sugar contents in sugar beets or in Nitrogen (N) is the nutrient most commonly poor quality malting barley. limiting for crop growth and development and is required in larger quantities by plants than any other mineral nutrient. -
Nitrogen Removal in Constructed Wetland Systems
Eng. Life Sci. 2009, 9, No. 1, 11–22 11 Chang-gyun Lee Review Tim D. Fletcher Guangzhi Sun Nitrogen removal in constructed wetland Department of Civil systems Engineering, Monash University, Victoria, Australia Since the mid 1990s, constructed wetlands have been increasingly used as a low- energy ‘green’ technique, in the treatment of wastewater and stormwater, driven by the rising cost of fossil fuels and increasing concern about climate change. Among various applications of these wetlands, a significant area is the removal of nitrogenous pollutants to protect the water environment and to enable effective reclamation and reuse of the wastewater. This paper provides a review of the current state of nitrogen removal technology, focusing on existing types of wetlands, the mechanisms of nitrogen removal, major environmental factors relative to nitrogen removal, and the operation and management of the wetlands. Keywords: Nitrogen removal / Reed bed / Subsurface flow / Wastewater treatment Received: April 13, 2008; revised: June 29, 2008; accepted: July 23, 2008 DOI: 10.1002/elsc.200800049 1 Introduction designed to simulate a natural wetland for waste treatment or other purposes [4]. In Europe, a constructed wetland is also Most wastewaters, such as industrial and agricultural wastewater, known as a reed bed. The evolution of constructed wetland urban drainage, sewage, and landfill leachate, contain nitrogen- technology has been comparatively short (the foundation was laid . ous compounds that have given rise to various negative by early researchers, notably two German scientists: Dr. Kathe phenomena in water environments, e.g. damage to aquatic life, Seidel and Dr. Reinhold Kickuth). Studies in this field were rare being toxic to fish and/or causing oxygen depletion in receiving until the second international conference on constructed wetlands water biota [1]. -
Ammoniacal Nitrogen (NH3-N) Is a Measure of the Amount of Ammonia
Ammoniacal nitrogen (NH3-N) is a measure of the amount of ammonia, a toxic pollutant often found in waste products, such as sewage, liquid manure and other liquid organic waste that can be toxic to aquatic life; elevated quantities in waterways are primarily from direct discharges of pollutants such as untreated effluent. Ammoniacal nitrogen levels are low in the River Witham both upstream and downstream of the Beck but were high in 2017 and 2018 in the Foston Beck in comparison to 2016, this may suggest a new source of ammoniacal nitrogen input into the drainage since January 2016 and may be a cause for concern warranting ongoing monitoring. Nitrate as nitrogen (NO3-N) is an important water quality metric used to indicate water pollution. Low levels of naturally occurring nitrate can be normal, but excess amounts can pollute groundwater. Common sources of nitrate in groundwater include fertilizers, livestock waste, and human waste associated with septic and municipal wastewater systems. Nitrate levels are higher in the Beck than in river and lower upstream of the Beck than downstream suggesting that the Beck is the source of raised nitrate levels. Values have not significantly changed in the three years of survey. Phosphates, as P, phosphates enter waterways from human and animal waste, phosphorus rich bedrock, laundry, cleaning, industrial effluents, and fertilizer runoff. These phosphates become detrimental when they over-fertilize aquatic plants causing eutrophication. Phosphate levels have oscillated in a similar pattern between all three sites between years but have generally been much lower in the Foston Beck than in the River Witham. -
Removal of Ammoniacal Nitrogen from Municipal
Facultad de Ciencias ACTA BIOLÓGICA COLOMBIANA Departamento de Biología http://www.revistas.unal.edu.co/index.php/actabiol Sede Bogotá ARTÍCULO DE INVESTIGACIÓN / RESEARCH ARTICLE BOTÁNICA REMOVAL OF AMMONIACAL NITROGEN FROM MUNICIPAL LANDFILL LEACHATE WITH FLOATING Typha domingensis (TYPHACEAE) Eliminación del nitrógeno amoniacal del lixiviado de vertedero municipal con Typha domingensis (TYPHACEAE) en flotación Camila Tamires PETRY¹ *, Daiane Trindade COSTA¹ , Annette DROSTE¹ 1Programa de Pós Graduação em Qualidade Ambiental, Universidade Feevale, ERS-239, 2755, Novo Hamburgo, Brasil *For correspondence: [email protected] Received: 06th September 2018, Returned for revision: 08th March 2019, Accepted: 02nd April 2019. Associate Editor: Sergi Sabater. Citation/Citar este artículo como: Petry CT, Costa DT, Droste A. Removal of ammoniacal nitrogen from municipal landfill leachate with floating Typha domingensis (Typhaceae). Acta biol. Colomb. 2020;25(1):5-13. DOI: http://dx.doi.org/10.15446/abc.v25n1.74749 ABSTRACT A promising method for the treatment of effluents is the use of floating macrophytes. Ammoniacal nitrogen is a typical compound present in urban landfill leachates and its removal is important due its toxicity to several organisms. Therefore, the study evaluated Typha domingensis survival and nitrification potential artificially floating in domestic solid waste leachate. Plants were exposed for 35 days to leachate (100, 75 and 50 %) and to rainwater with N:P:K (control). Dissolved oxygen (DO) of the treatments was periodically measured, and ammoniacal nitrogen, nitrite and nitrate were analyzed before and after exposure. At the end of the experiment, plant survival rate was calculated. After two weeks, DO increased twice in the control, three times in 50 % leachate, four times in 75 % leachate, and eight times in 100 % leachate.