Molecular Aspects of Bacterial Nanocellulose Biosynthesis
Total Page:16
File Type:pdf, Size:1020Kb
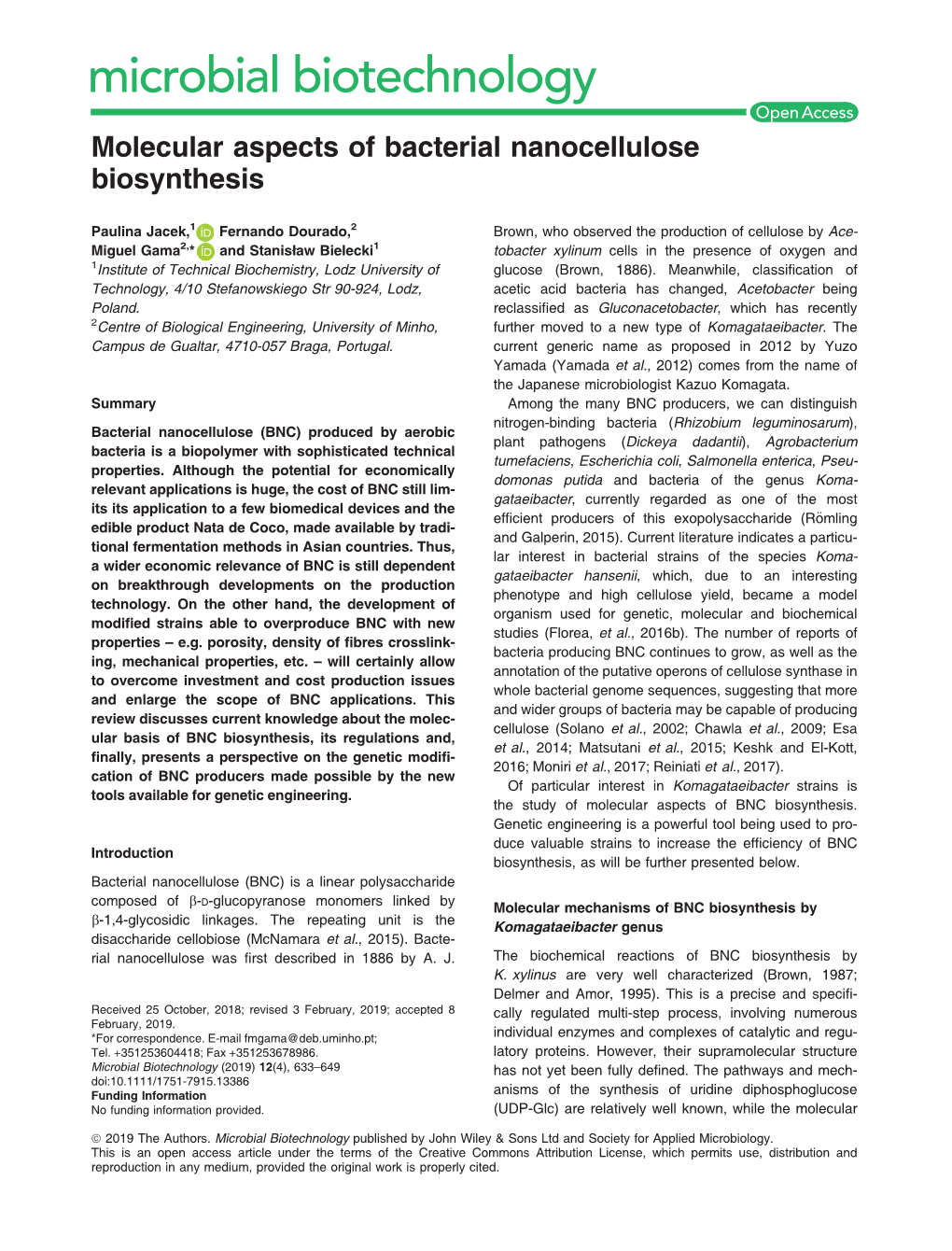
Load more
Recommended publications
-
Kombucha Tea As a Reservoir of Cellulose Producing Bacteria: Assessing Diversity Among Komagataeibacter Isolates
applied sciences Article Kombucha Tea as a Reservoir of Cellulose Producing Bacteria: Assessing Diversity among Komagataeibacter Isolates Salvatore La China , Luciana De Vero , Kavitha Anguluri, Marcello Brugnoli, Dhouha Mamlouk and Maria Gullo * Department of Life Sciences, University of Modena and Reggio Emilia, 42122 Reggio Emilia, Italy; [email protected] (S.L.C.); [email protected] (L.D.V.); [email protected] (K.A.); [email protected] (M.B.); [email protected] (D.M.) * Correspondence: [email protected] Abstract: Bacterial cellulose (BC) is receiving a great deal of attention due to its unique properties such as high purity, water retention capacity, high mechanical strength, and biocompatibility. However, the production of BC has been limited because of the associated high costs and low productivity. In light of this, the isolation of new BC producing bacteria and the selection of highly productive strains has become a prominent issue. Kombucha tea is a fermented beverage in which the bacteria fraction of the microbial community is composed mostly of strains belonging to the genus Komagataeibacter. In this study, Kombucha tea production trials were performed starting from a previous batch, and bacterial isolation was conducted along cultivation time. From the whole microbial pool, 46 isolates were tested for their ability to produce BC. The obtained BC yield ranged from 0.59 g/L, for the isolate K2G36, to 23 g/L for K2G30—which used as the reference strain. The genetic intraspecific diversity of the 46 isolates was investigated using two repetitive-sequence-based PCR typing methods: the enterobacterial repetitive intergenic consensus (ERIC) elements and the (GTG)5 sequences, Citation: La China, S.; De Vero, L.; respectively. -
Embracing Bacterial Cellulose As a Catalyst for Sustainable Fashion
Running head: BACTERIAL CELLULOSE 1 Embracing Bacterial Cellulose as a Catalyst for Sustainable Fashion Luis Quijano A Senior Thesis submitted in partial fulfillment of the requirements for graduation in the Honors Program Liberty University Fall 2017 BACTERIAL CELLULOSE 2 Acceptance of Senior Honors Thesis This Senior Honors Thesis is accepted in partial fulfillment of the requirements for graduation from the Honors Program of Liberty University. ______________________________ Debbie Benoit, D.Min. Thesis Chair ______________________________ Randall Hubbard, Ph.D. Committee Member ______________________________ Matalie Howard, M.S. Committee Member ______________________________ David E. Schweitzer, Ph.D. Assistant Honors Director ______________________________ Date BACTERIAL CELLULOSE 3 Abstract Bacterial cellulose is a leather-like material produced during the production of Kombucha as a pellicle of bacterial cellulose (SCOBY) using Kombucha SCOBY, water, sugar, and green tea. Through an examination of the bacteria that produces the cellulose pellicle of the interface of the media and the air, currently named Komagataeibacter xylinus, an investigation of the growing process of bacterial cellulose and its uses, an analysis of bacterial cellulose’s properties, and a discussion of its prospects, one can fully grasp bacterial cellulose’s potential in becoming a catalyst for sustainable fashion. By laying the groundwork for further research to be conducted in bacterial cellulose’s applications as a textile, further commercialization of bacterial cellulose may become a practical reality. Keywords: Acetobacter xylinum, alternative textile, bacterial cellulose, fashion, Komagateibacter xylinus, sustainability BACTERIAL CELLULOSE 4 Embracing Bacterial Cellulose as a Catalyst for Sustainable Fashion Introduction One moment the fashionista is “in”, while the next day the fashionista is “out”. The fashion industry is typically known for its ever-changing trends, styles, and outfits. -
A High Bacterial Cellulose Producing Strain in Glucose and Mannitol Based Media
fmicb-10-00058 January 29, 2019 Time: 16:59 # 1 ORIGINAL RESEARCH published: 30 January 2019 doi: 10.3389/fmicb.2019.00058 Exploring K2G30 Genome: A High Bacterial Cellulose Producing Strain in Glucose and Mannitol Based Media Maria Gullo1*, Salvatore La China1, Giulio Petroni2, Simona Di Gregorio2 and Paolo Giudici1 1 Department of Life Sciences, University of Modena and Reggio Emilia, Reggio Emilia, Italy, 2 Department of Biology, University of Pisa, Pisa, Italy Demands for renewable and sustainable biopolymers have rapidly increased in the last decades along with environmental issues. In this context, bacterial cellulose, as renewable and biodegradable biopolymer has received considerable attention. Particularly, acetic acid bacteria of the Komagataeibacter xylinus species can produce bacterial cellulose from several carbon sources. To fully exploit metabolic potential of cellulose producing acetic acid bacteria, an understanding of the ability of producing Edited by: bacterial cellulose from different carbon sources and the characterization of the Cinzia Caggia, genes involved in the synthesis is required. Here, K2G30 (UMCC 2756) was studied Università degli Studi di Catania, Italy with respect to bacterial cellulose production in mannitol, xylitol and glucose media. Reviewed by: Moreover, the draft genome sequence with a focus on cellulose related genes was Hubert Antolak, Lodz University of Technology, Poland produced. A pH reduction and gluconic acid formation was observed in glucose medium Lidia Stasiak, which allowed to produce 6.14 ± 0.02 g/L of bacterial cellulose; the highest bacterial Warsaw University of Life Sciences, Poland cellulose production obtained was in 1.5% (w/v) mannitol medium (8.77 ± 0.04 g/L), *Correspondence: while xylitol provided the lowest (1.35 ± 0.05 g/L) yield. -
Kombucha: a Novel Model System for Cooperation and Conflict in A
Kombucha: a novel model system for cooperation and conflict in a complex multi-species microbial ecosystem Alexander May1,2, Shrinath Narayanan3, Joe Alcock4, Arvind Varsani1,5,6,7, Carlo Maley1,3 and Athena Aktipis2,3,5,7 1 School of Life Sciences, Arizona State University, Tempe, AZ, USA 2 Department of Psychology, Arizona State University, Tempe, AZ, USA 3 The Biodesign Center for Biocomputing, Security and Society, Arizona State University, Tempe, AZ, USA 4 University of New Mexico, Albuquerque, NM, USA 5 The Biodesign Center for Fundamental and Applied Microbiomics, Center for Evolution and Medicine, School of Life Sciences, Arizona State University, Tempe, AZ, USA 6 Structural Biology Research Unit, Department of Clinical Laboratory Sciences, University of Cape Town, Cape Town, South Africa 7 Center for Evolution and Medicine, Arizona State University, Tempe, AZ, USA ABSTRACT Kombucha, a fermented tea beverage with an acidic and effervescent taste, is composed of a multispecies microbial ecosystem with complex interactions that are characterized by both cooperation and conflict. In kombucha, a complex community of bacteria and yeast initiates the fermentation of a starter tea (usually black or green tea with sugar), producing a biofilm that covers the liquid over several weeks. This happens through several fermentative phases that are characterized by cooperation and competition among the microbes within the kombucha solution. Yeast produce invertase as a public good that enables both yeast and bacteria to metabolize sugars. Bacteria produce a surface biofilm which may act as a public good providing protection from invaders, storage for resources, and greater access to oxygen for microbes embedded within it. -
Table S5. the Information of the Bacteria Annotated in the Soil Community at Species Level
Table S5. The information of the bacteria annotated in the soil community at species level No. Phylum Class Order Family Genus Species The number of contigs Abundance(%) 1 Firmicutes Bacilli Bacillales Bacillaceae Bacillus Bacillus cereus 1749 5.145782459 2 Bacteroidetes Cytophagia Cytophagales Hymenobacteraceae Hymenobacter Hymenobacter sedentarius 1538 4.52499338 3 Gemmatimonadetes Gemmatimonadetes Gemmatimonadales Gemmatimonadaceae Gemmatirosa Gemmatirosa kalamazoonesis 1020 3.000970902 4 Proteobacteria Alphaproteobacteria Sphingomonadales Sphingomonadaceae Sphingomonas Sphingomonas indica 797 2.344876284 5 Firmicutes Bacilli Lactobacillales Streptococcaceae Lactococcus Lactococcus piscium 542 1.594633558 6 Actinobacteria Thermoleophilia Solirubrobacterales Conexibacteraceae Conexibacter Conexibacter woesei 471 1.385742446 7 Proteobacteria Alphaproteobacteria Sphingomonadales Sphingomonadaceae Sphingomonas Sphingomonas taxi 430 1.265115184 8 Proteobacteria Alphaproteobacteria Sphingomonadales Sphingomonadaceae Sphingomonas Sphingomonas wittichii 388 1.141545794 9 Proteobacteria Alphaproteobacteria Sphingomonadales Sphingomonadaceae Sphingomonas Sphingomonas sp. FARSPH 298 0.876754244 10 Proteobacteria Alphaproteobacteria Sphingomonadales Sphingomonadaceae Sphingomonas Sorangium cellulosum 260 0.764953367 11 Proteobacteria Deltaproteobacteria Myxococcales Polyangiaceae Sorangium Sphingomonas sp. Cra20 260 0.764953367 12 Proteobacteria Alphaproteobacteria Sphingomonadales Sphingomonadaceae Sphingomonas Sphingomonas panacis 252 0.741416341 -
Missense Mutations in a Transmembrane Domain of The
Salgado et al. BMC Microbiology (2019) 19:216 https://doi.org/10.1186/s12866-019-1577-5 RESEARCH ARTICLE Open Access Missense mutations in a transmembrane domain of the Komagataeibacter xylinus BcsA lead to changes in cellulose synthesis Luis Salgado1, Silvia Blank2, Reza Alipour Moghadam Esfahani1, Janice L. Strap1 and Dario Bonetta1* Abstract Background: Cellulose is synthesized by an array of bacterial species. Komagataeibacter xylinus is the best characterized as it produces copious amounts of the polymer extracellularly. Despite many advances in the past decade, the mechanisms underlying cellulose biosynthesis are not completely understood. Elucidation of these mechanisms is essential for efficient cellulose production in industrial applications. Results: In an effort to gain a better understanding of cellulose biosynthesis and its regulation, cellulose crystallization was investigated in K. xylinus mutants resistant to an inhibitor of cellulose I formation, pellicin. Through the use of forward genetics and site-directed mutagenesis, A449T and A449V mutations in the K. xylinus BcsA protein were found to be important for conferring high levels of pellicin resistance. Phenotypic analysis of the bcsAA449T and bcsAA449V cultures revealed that the mutations affect cellulose synthesis rates and that cellulose crystallinity is affected in wet pellicles but not dry ones. Conclusions: A449 is located in a predicted transmembrane domain of the BcsA protein suggesting that the structure of the transmembrane domain influences cellulose crystallization either by affecting the translocation of the nascent glucan chain or by allosterically altering protein-protein interactions. Keywords: Komagataeibacter xylinus, Pellicin, BcsA, Crystallinity Background types of allomorphs; with the Iβ form dominant in higher Cellulose, an abundant glucan polymer, is synthesized by plants and the Iα form dominant in bacteria and algal spe- organisms belonging to a variety of phylogenetic cies [7]. -
Bacterial Nanocellulose: What Future? Francisco Miguel Portela Da Gama*, Fernando Dourado
Portela da Gama FM., Dourado F., BioImpacts, 2018, 8(1), 1-3 doi: 10.15171/bi.2018.01 TUOMS Publishing BioImpacts http://bi.tbzmed.ac.ir/ Group Publish Free ccess Bacterial NanoCellulose: what future? Francisco Miguel Portela da Gama*, Fernando Dourado Centre of Biological Engineering, University of Minho, Campus de Gualtar 4710-057 Braga, Portugal Article Info Summary Authors' Biosketch Miguel Gama (Ph.D.) is Acetic acid bacteria (AAB) have been used in an Associate Professor various fermentation processes. Of several ABB, the with Habilitation at Minho bacterial nanocellulose (BNC) producers, notably University, where he develops his Academic Komagataeibacter xylinus, appears as an interesting career since 1992. His species, in large part because of their ability in the current research interests include (i) bacterial secretion of cellulose as nano/microfibrils. In fact, nanocellulose production BNC is characterized by a native nanofibrillar and its applications in food technology and the Article Type: structure, which may outperform the currently biomedical field, (ii) development of self-assembled Editorial nanogels made of polysaccharides, (iii) the production used celluloses in the food industry as a promising and characterization of the biomaterials, the novel hydrocolloid additive. During the last couple development of drug delivery systems for antimicrobial Article History: of years, a number of companies worldwide have peptides and low molecular weight hydrophobic drugs. Received: 10 Dec. 2017 Prof. Gama is currently Vice-Director of the Centre Accepted: 15 Dec. 2017 introduced some BNC-based products to the market. of Biological Engineering (2016-ongoing), has been The main aim of this editorial is to underline the Director of the Biomedical Engineering Integrated ePublished: 15 Dec. -
Mechanical Properties of Bacterial Cellulose Synthesised by Diverse Strains of the Genus Komagataeibacter
Accepted Manuscript Mechanical properties of bacterial cellulose synthesised by diverse strains of the genus Komagataeibacter Si-Qian Chen, Patricia Lopez-Sanchez, Dongjie Wang, Deirdre Mikkelsen, Michael J. Gidley PII: S0268-005X(17)31582-5 DOI: 10.1016/j.foodhyd.2018.02.031 Reference: FOOHYD 4290 To appear in: Food Hydrocolloids Received Date: 29 September 2017 Revised Date: 22 December 2017 Accepted Date: 18 February 2018 Please cite this article as: Chen, S.-Q., Lopez-Sanchez, P., Wang, D., Mikkelsen, D., Gidley, M.J., Mechanical properties of bacterial cellulose synthesised by diverse strains of the genus Komagataeibacter, Food Hydrocolloids (2018), doi: 10.1016/j.foodhyd.2018.02.031. This is a PDF file of an unedited manuscript that has been accepted for publication. As a service to our customers we are providing this early version of the manuscript. The manuscript will undergo copyediting, typesetting, and review of the resulting proof before it is published in its final form. Please note that during the production process errors may be discovered which could affect the content, and all legal disclaimers that apply to the journal pertain. ACCEPTED MANUSCRIPT Diverse mechanical properties of bacterial cellulose hydrogels determined by cellulose concentration and fibril architecture Oscillation Extension Compression/relaxation MANUSCRIPT ACCEPTED 1 Mechanical properties of Bacterial Cellulose Synthesised by Diverse Strains of the 2 Genus Komagataeibacter 3 4 Si-Qian Chen 1, Patricia Lopez-Sanchez 1, Dongjie Wang 1, #, Deirdre Mikkelsen 1 and Michael 5 J Gidley 1* 6 7 1 ARC Centre of Excellence in Plant Cell Walls, Centre for Nutrition and Food Sciences, 8 Queensland Alliance for Agriculture and Food Innovation, The University of Queensland, St. -
Bacterial Diversity Shift Determined by Different Diets in the Gut of the Spotted Wing Fly Drosophila Suzukii Is Primarily Reflected on Acetic Acid Bacteria
Bacterial diversity shift determined by different diets in the gut of the spotted wing fly Drosophila suzukii is primarily reflected on acetic acid bacteria Item Type Article Authors Vacchini, Violetta; Gonella, Elena; Crotti, Elena; Prosdocimi, Erica M.; Mazzetto, Fabio; Chouaia, Bessem; Callegari, Matteo; Mapelli, Francesca; Mandrioli, Mauro; Alma, Alberto; Daffonchio, Daniele Citation Vacchini V, Gonella E, Crotti E, Prosdocimi EM, Mazzetto F, et al. (2016) Bacterial diversity shift determined by different diets in the gut of the spotted wing fly Drosophila suzukii is primarily reflected on acetic acid bacteria. Environmental Microbiology Reports. Available: http://dx.doi.org/10.1111/1758-2229.12505. Eprint version Post-print DOI 10.1111/1758-2229.12505 Publisher Wiley Journal Environmental Microbiology Reports Rights This is the peer reviewed version of the following article: Vacchini, V., Gonella, E., Crotti, E., Prosdocimi, E. M., Mazzetto, F., Chouaia, B., Callegari, M., Mapelli, F., Mandrioli, M., Alma, A. and Daffonchio, D. (2016), Bacterial diversity shift determined by different diets in the gut of the spotted wing fly Drosophila suzukii is primarily reflected on acetic acid bacteria. Environmental Microbiology Reports. Accepted Author Manuscript. doi:10.1111/1758-2229.12505, which has been published in final form at http://onlinelibrary.wiley.com/ doi/10.1111/1758-2229.12505/abstract. This article may be used for non-commercial purposes in accordance With Wiley Terms and Conditions for self-archiving. Download date 02/10/2021 16:15:24 Link to Item http://hdl.handle.net/10754/622736 Bacterial diversity shift determined by different diets in the gut of the spotted wing fly Drosophila suzukii is primarily reflected on acetic acid bacteria Violetta Vacchini1#, Elena Gonella2#, Elena Crotti1#, Erica M. -
An Expanded Synthetic Biology Toolkit for Gene Expression Control in Acetobacteraceae
This document is downloaded from DR‑NTU (https://dr.ntu.edu.sg) Nanyang Technological University, Singapore. An expanded synthetic biology toolkit for gene expression control in Acetobacteraceae Teh, Min Yan; Ooi, Kean Hean; Teo, Danny Shun Xiang; Mohammad Ehsan Mansoor; Lim, Shaun Wen Zheng; Tan, Meng How 2019 Teh, M. Y., Ooi, K. H., Teo, D. S. X., Mohammad Ehsan Mansoor, Lim, S. W. Z., & Tan, M. H. (2019). An expanded synthetic biology toolkit for gene expression control in Acetobacteraceae. ACS Synthetic Biology, 8(4), 708‑723. doi:10.1021/acssynbio.8b00168 https://hdl.handle.net/10356/144734 https://doi.org/10.1021/acssynbio.8b00168 This document is the Accepted Manuscript version of a Published Work that appeared in final form in ACS Synthetic Biology, copyright © American Chemical Society after peer review and technical editing by the publisher. To access the final edited and published work see https://doi.org/10.1021/acssynbio.8b00168 Downloaded on 27 Sep 2021 03:08:06 SGT An expanded synthetic biology toolkit for gene expression control in Acetobacteraceae Min Yan Teh1,4, Kean Hean Ooi1,2,4, Shun Xiang Danny Teo1,2,3, Mohammad Ehsan Bin Mansoor1, Wen Zheng Shaun Lim1, Meng How Tan1,3 1School of Chemical and Biomedical Engineering, Nanyang Technological University, Singapore 637459, Singapore 2School of Biological Sciences, Nanyang Technological University, Singapore 637551, Singapore 3Genome Institute of Singapore, Agency for Science Technology and Research, Singapore 138672, Singapore 4These authors contributed equally to this work. *Correspondence: [email protected] or [email protected] 1 ABSTRACT The availability of different host chassis will greatly expand the range of applications in synthetic biology. -
Proposal Form to Prescribe Certain Organisms As Not New Organisms for the Purposes of the Hazardous Substances and New Organisms (HSNO) Act
PROPOSAL FORM Proposal form to prescribe certain organisms as not new organisms for the purposes of the Hazardous Substances and New Organisms (HSNO) Act Send to the Environmental Protection Authority preferably by email [email protected] or alternatively by post to: Private Bag 63002, Wellington 6140 Name of person or organisation making the proposal SCION Postal Address Te Papa Tipu Innovation Park. 49 Sala Street, Rotorua 3010. Private Bag 3020 Date 12 September 2016 www.epa.govt.nz 2 Proposal Form Important If species were not present in New Zealand before 29 July 1998, they are classed as new organisms under the Hazardous Substances and New Organisms (HSNO) Act. As such, they will require HSNO Act approval for propagation or distribution of the organism to occur. Currently, if anyone was to conduct any of these activities without a HSNO Act approval they would be committing an offence under section 109(1) of the Act. To change its “new organism” status (which means that an organism will not be regulated under the HSNO Act), an organism must be deregulated under section 140(1)(ba) of the HSNO Act, by an Order in Council given by the Governor General prescribing organisms that are not new organisms for the purposes of this Act. As part of this process, the following form is to be filled in by the person or organisation making a proposal to prescribe certain new organisms as not new organisms. The information provided in this form will be used in the decision-making process (which is likely to include a public consultation component). -
Potato Juice, a Starch Industry Waste, As a Cost-Effective Medium for the Biosynthesis of Bacterial Cellulose
bioRxiv preprint doi: https://doi.org/10.1101/2021.07.15.452442; this version posted July 15, 2021. The copyright holder for this preprint (which was not certified by peer review) is the author/funder, who has granted bioRxiv a license to display the preprint in perpetuity. It is made available under aCC-BY-NC-ND 4.0 International license. Potato juice, a starch industry waste, as a cost-effective medium for the biosynthesis of bacterial cellulose Daria Ciecholewska-Juśkoa, Michał Brodaa,b, Anna Żywickaa, Daniel Styburskic, Peter Sobolewskid, Krzysztof Gorącyd, Paweł Migdałe, Adam Junkaf, Karol Fijałkowskia* a Department of Microbiology and Biotechnology, Faculty of Biotechnology and Animal Husbandry, West Pomeranian University of Technology, Szczecin, Piastów 45, 70-311 Szczecin, Poland; [email protected]; [email protected]; [email protected]; [email protected] b Pomeranian-Masurian Potato Breeding Company, 76-024, Strzekęcino, Poland; c Laboratory of Chromatography and Mass Spectroscopy, Faculty of Biotechnology and Animal Husbandry, West Pomeranian University of Technology, Szczecin, Klemensa Janickiego 29, 71-270, Szczecin, Poland; [email protected] d Department of Polymer and Biomaterials Science, Faculty of Chemical Technology and Engineering, West Pomeranian University of Technology, Szczecin, Piastów 45, 70-311 Szczecin, Poland; [email protected]; [email protected] e Department of Environment, Hygiene and Animal Welfare, Faculty of Biology and Animal Science, Wroclaw University of Environmental and Life Sciences, Chełmońskiego 38C, 51-630 Wrocław, Poland; [email protected] f Department of Pharmaceutical Microbiology and Parasitology, Faculty of Pharmacy, Medical University of Wroclaw, Borowska 211a, 50-534 Wrocław, Poland; [email protected] Corresponding author E-mail address: [email protected] (K.