Identification and Manipulation of Memory Engram Cells
Total Page:16
File Type:pdf, Size:1020Kb
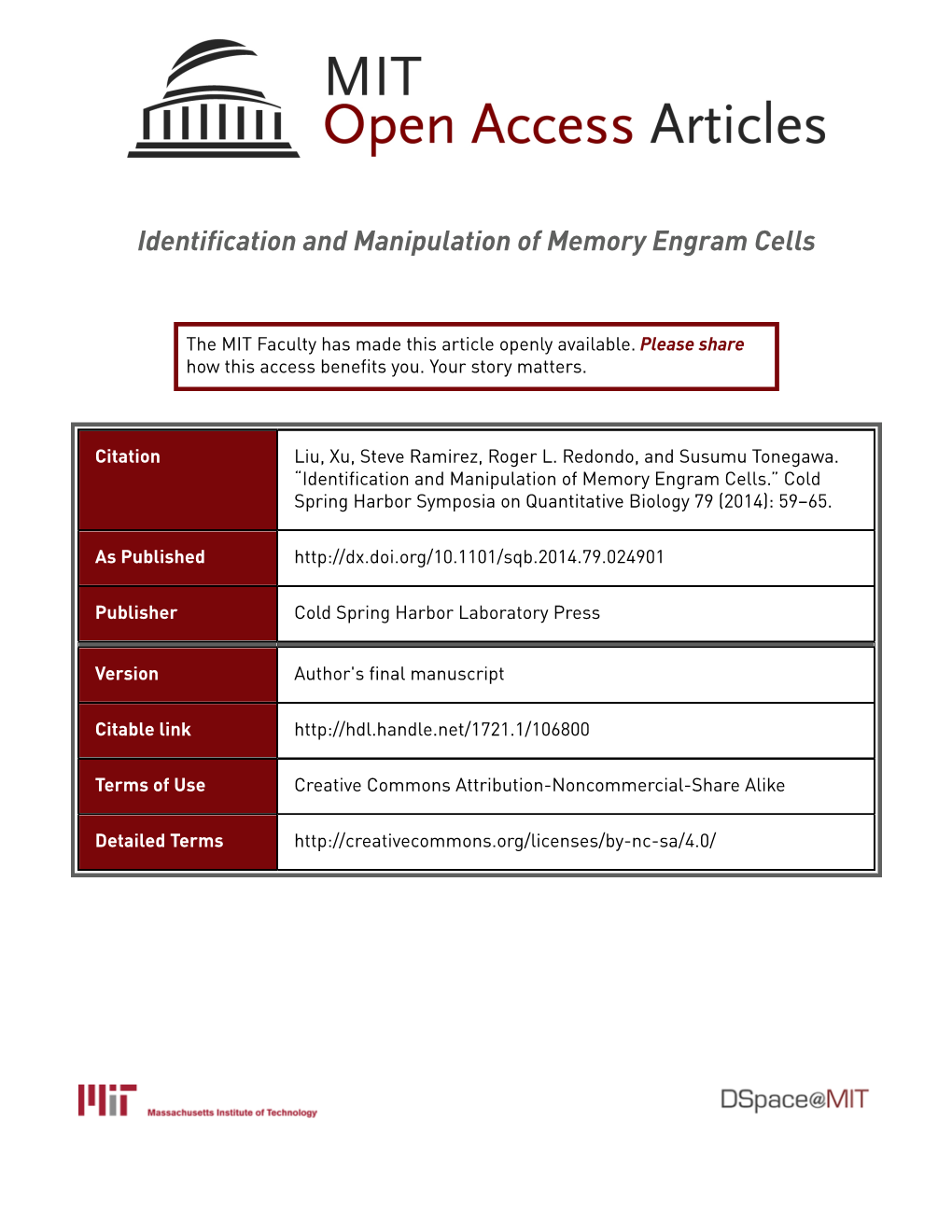
Load more
Recommended publications
-
Dynamics of Memory Engrams
G Model NSR-4275; No. of Pages 5 ARTICLE IN PRESS Neuroscience Research xxx (2019) xxx–xxx Contents lists available at ScienceDirect Neuroscience Research jo urnal homepage: www.elsevier.com/locate/neures Update Article Dynamics of memory engrams ∗ Shogo Takamiya, Shoko Yuki, Junya Hirokawa, Hiroyuki Manabe, Yoshio Sakurai Laboratory of Neural Information, Graduate School of Brain Science, Doshisha University, Kyotanabe 610-0394, Kyoto, Japan a r t i c l e i n f o a b s t r a c t Article history: In this update article, we focus on “memory engrams”, which are traces of long-term memory in the brain, Received 21 February 2019 and emphasizes that they are not static but dynamic. We first introduce the major findings in neuroscience Received in revised form 18 March 2019 and psychology reporting that memory engrams are sometimes diffuse and unstable, indicating that Accepted 27 March 2019 they are dynamically modified processes of consolidation and reconsolidation. Second, we introduce and Available online xxx discuss the concepts of cell assembly and engram cell, the former has been investigated by psychological experiments and behavioral electrophysiology and the latter is defined by recent combination of activity- Keywords: dependent cell labelling with optogenetics to show causal relationships between cell population activity Memory engram and behavioral changes. Third, we discuss the similarities and differences between the cell assembly and Memory reconsolidation engram cell concepts to reveal the dynamics of memory engrams. We also discuss the advantages and Cell assembly Engram cell problems of live-cell imaging, which has recently been developed to visualize multineuronal activities. -
On the Trail of the Hippocampal Engram
Physiological Psychology 1980, Vol. 8 (2),229-238 On the trail of the hippocampal engram JOHN O'KEEFE and DULCIE H. CONWAY Cerebral Functions Group, Anatomy Department and Centre/or Neuroscience University College London, London WCIE 6BT, England Recent ideas about hippocampal function in animals suggest that it may be involved in memory. A new test for rat memory is described (the despatch task). It has several properties which make it well suited for the study of one-trial, long-term memory. The information about the location of the goal is given to the rat when it is in the startbox. The memory formed is long lasting (> 30 min), and the animals are remembering the location of the goal and not the response to be made to reach that goal. The spatial relations of the cues within the environ ment can be manipulated to bias the animals towards selection of a place hypothesis or a guidance hypothesis. The latter does not seem to support long-term memory formation in the way that the former does. Fornix lesions selectively disrupt performance of the place-biased task, a finding that is predicted by the cognitive map theory but not by other ideas about hippocampal involvement in memory. Since 1957, when Scoville and Milner (1957) reported is little interference between different place representa that bilateral removal of the mesial temporal lobes in tions. Consequently, the mapping system should be man resulted in a profound global amnesia, it has gener capable of supporting high levels of performance in tests ally been accepted that the hippocampus plays an of animal memory, such as the delayed reaction task important role in human memory (but see Horel, 1978, of Hunter (1913)- for an important paper questioning this dogma). -
Searching for the Memory New Research Sheds Light—Literally—On Recall Mechanisms by Christof Koch
(consciousness redux) Searching for the Memory New research sheds light—literally—on recall mechanisms BY CHRISTOF KOCH The difference between false gion as far as learning is concerned. page 76], and you will not be able to memories and true ones is the The most singular feature of science form new explicit memories, whereas same as for jewels: it is always the that distinguishes it from other human losses of large swaths of visual cortex false ones that look the most real, activities, such as art or religion, and leave the subjects blind but without the most brilliant. gives it a dynamics of its own, is prog- memory impairments. Yet percepts and memo- THIS QUOTE by surrealist ries are not born of brain re- painter Salvador Dalí comes gions but arise within intri- to mind when pondering the cate networks of neurons, latest wizardry coming out of connected by synapses. Neu- two neurobiology laborato- rons, rather than chunks of ries. Before we come to that, brain, are the atoms of however, let us remember thoughts, consciousness and that ever since Plato and Aris- remembering. totle first likened memories to impressions made onto wax Implanting a False tablets, philosophers and nat- Memory in Mice ural scientists have searched If you have ever been the for the physical substrate of victim of a mugging in a des- memories. In the first half of olate parking garage, you the 20th-century psycholo- may carry that occurrence gists carried out carefully with you to the end of your controlled experiments to days. Worse, whenever you look for the so-called memo- walk into a parking struc- ry engram in the brain. -
Universal Memory Mechanism for Familiarity Recognition and Identification
The Journal of Neuroscience, January 2, 2008 • 28(1):239–248 • 239 Behavioral/Systems/Cognitive Universal Memory Mechanism for Familiarity Recognition and Identification Volodya Yakovlev,1 Daniel J. Amit,2,3† Sandro Romani,4 and Shaul Hochstein1 1Life Sciences Institute & Neural Computation Center and 2Racah Institute of Physics, Hebrew University, Jerusalem 91904, Israel, and 3Department of Physics and 4Doctoral Program in Neurophysiology, Department of Human Physiology, University of Rome “La Sapienza,” 00185 Rome, Italy Macaque monkeys were tested on a delayed-match-to-multiple-sample task, with either a limited set of well trained images (in random- ized sequence) or with never-before-seen images. They performed much better with novel images. False positives were mostly limited to catch-trial image repetitions from the preceding trial. This result implies extremely effective one-shot learning, resembling Standing’s finding that people detect familiarity for 10,000 once-seen pictures (with 80% accuracy) (Standing, 1973). Familiarity memory may differ essentially from identification, which embeds and generates contextual information. When encountering another person, we can say immediately whether his or her face is familiar. However, it may be difficult for us to identify the same person. To accompany the psychophysical findings, we present a generic neural network model reproducing these behaviors, based on the same conservative Hebbian synaptic plasticity that generates delay activity identification memory. Familiarity becomes the first step toward establishing identification. Adding an inter-trial reset mechanism limits false positives for previous-trial images. The model, unlike previous propos- als, relates repetition–recognition with enhanced neural activity, as recently observed experimentally in 92% of differential cells in prefrontal cortex, an area directly involved in familiarity recognition. -
Memory Engram Storage and Retrieval
Available online at www.sciencedirect.com ScienceDirect Memory engram storage and retrieval 1,2 1 1 Susumu Tonegawa , Michele Pignatelli , Dheeraj S Roy and 1,2 Toma´ s J Ryan A great deal of experimental investment is directed towards likely distributed nature of a memory engram throughout questions regarding the mechanisms of memory storage. Such the brain [6 ]. Here we review recent experimental stud- studies have traditionally been restricted to investigation of the ies on the identification of memory engram cells, with a anatomical structures, physiological processes, and molecular focus on the mechanisms of memory storage. A more pathways necessary for the capacity of memory storage, and comprehensive review of recent memory engram studies have avoided the question of how individual memories are is available elsewhere [7 ]. stored in the brain. Memory engram technology allows the labeling and subsequent manipulation of components of Memory function and the hippocampus specific memory engrams in particular brain regions, and it has The medial temporal lobe (MTL), in particular the hip- been established that cell ensembles labeled by this method are both sufficient and necessary for memory recall. Recent pocampus, was implicated in memory of events or episodes research has employed this technology to probe fundamental by neurological studies of human clinical patients, where questions of memory consolidation, differentiating between its direct electrophysiological stimulation evoked the recall mechanisms of memory retrieval from the -
What Is Memory? the Present State of the Engram Mu-Ming Poo1*, Michele Pignatelli2, Tomás J
Poo et al. BMC Biology (2016)14:40 DOI 10.1186/s12915-016-0261-6 FORUM Open Access What is memory? The present state of the engram Mu-ming Poo1*, Michele Pignatelli2, Tomás J. Ryan2,3, Susumu Tonegawa2,3*, Tobias Bonhoeffer4, Kelsey C. Martin5, Andrii Rudenko6, Li-Huei Tsai6, Richard W. Tsien7, Gord Fishell7, Caitlin Mullins7, J. Tiago Gonçalves8, Matthew Shtrahman8, Stephen T. Johnston8, Fred H. Gage8, Yang Dan9, John Long7, György Buzsáki7 and Charles Stevens8 connections due to correlated activities during memory ac- Abstract quisition. The discovery of activity-induced long-term po- The mechanism of memory remains one of the great tentiation (LTP) and long-term depression (LTD) of central unsolved problems of biology. Grappling with the synapses in the 1970s and 80s further sparked the interest of question more than a hundred years ago, the German a whole generation of neurobiologists in studying synaptic zoologist Richard Semon formulated the concept of plasticity and its relationship to memory. There is now gen- the engram, lasting connections in the brain that eral consensus that persistent modification of the synaptic result from simultaneous “excitations”, whose precise strength via LTP and LTD of pre-existing connections physical nature and consequences were out of reach represents a primary mechanism for the formation of mem- of the biology of his day. Neuroscientists now have ory engrams. In addition, LTP and LTD could also lead to the knowledge and tools to tackle this question, the formation of new and elimination of old synapses and however, and this Forum brings together leading thus changes in structural connectivity in the brain. -
From Engrams to Pathologies of the Brain
REVIEW published: 07 April 2017 doi: 10.3389/fncir.2017.00023 From Engrams to Pathologies of the Brain Christine A. Denny 1,2, Evan Lebois 3 and Steve Ramirez 4* 1Department of Psychiatry, Columbia University, New York, NY, USA, 2Division of Integrative Neuroscience, New York State Psychiatric Institute (NYSPI)/Research Foundation for Mental Hygiene, Inc. (RFMH), New York, NY, USA, 3Neuroscience and Pain Research Unit, Pfizer Inc., Cambridge, MA, USA, 4Center for Brain Science, Harvard University, Cambridge, MA, USA Memories are the experiential threads that tie our past to the present. The biological realization of a memory is termed an engram—the enduring biochemical and physiological processes that enable learning and retrieval. The past decade has witnessed an explosion of engram research that suggests we are closing in on boundary conditions for what qualifies as the physical manifestation of memory. In this review, we provide a brief history of engram research, followed by an overview of the many rodent models available to probe memory with intersectional strategies that have yielded unprecedented spatial and temporal resolution over defined sets of cells. We then discuss the limitations and controversies surrounding engram research and subsequently attempt to reconcile many of these views both with data and by proposing a conceptual shift in the strategies utilized to study memory. We finally bridge this literature with human memory research and disorders of the brain and end by providing an experimental blueprint for future engram studies in mammals. Collectively, we believe that we are in an era of neuroscience where engram research has transitioned from ephemeral and philosophical concepts to provisional, tractable, experimental frameworks for studying the cellular, circuit and behavioral manifestations Edited by: of memory. -
Finding the Engram
REVIEWS Finding the engram Sheena A. Josselyn1–4, Stefan Köhler5,6 and Paul W. Frankland1–4 Abstract | Many attempts have been made to localize the physical trace of a memory, or engram, in the brain. However, until recently, engrams have remained largely elusive. In this Review, we develop four defining criteria that enable us to critically assess the recent progress that has been made towards finding the engram. Recent ‘capture’ studies use novel approaches to tag populations of neurons that are active during memory encoding, thereby allowing these engram-associated neurons to be manipulated at later times. We propose that findings from these capture studies represent considerable progress in allowing us to observe, erase and express the engram. Neuronal ensembles Memories are thought to be encoded as enduring physi memory in the brain. Semon suggested that an engram 1,2 9 Collections of neurons that cal changes in the brain, or engrams . Most neuro has four defining characteristics (FIG. 1). First, an engram is show coordinated firing scientists agree that the formation of an engram involves a persistent change in the brain that results from a specific activity, equivalent to the cell strengthening of synaptic connections between popula experience or event. Second, an engram has the potential assembly defined by Hebb. tions of neurons (neuronal ensembles). However, charac for ecphory; that is, an engram may be expressed behav terizing the precise nature and location of engrams has iourally through interactions with retrieval cues, which been challenging. Lashley was among the first to attempt could be sensory input, ongoing behaviour or voluntary to localize engrams using an empirical approach3,4. -
To Master Memory, Researchers Pursue Its Roots Novel Approaches Have Reinvigorated the Search for the Elusive Memory Engram—And Energized Attempts to Control It
NEWS FEATURE NEWS FEATURE To master memory, researchers pursue its roots Novel approaches have reinvigorated the search for the elusive memory engram—and energized attempts to control it. Helen H. Shen, Science Writer What is a memory? Can it be purposefully erased, had actually occurred (1). This work was a powerful implanted, or altered? Such musings have long cap- demonstration of the access and control that neurosci- tured the public imagination, inspiring cinematic tales entists are gaining over the once-elusive engram, the of memory manipulation, ranging from the political physical embodiment of a memory in the brain. thriller The Manchurian Candidate, to the sci-fi classic Ever since the idea was first formalized in the early Total Recall. In the quirky romance Eternal Sunshine of 1900s, researchers have struggled to capture and the Spotless Mind, memory technicians erase painful elucidate the engram. Neuroscientists theorized that recollections of failed relationships. In Inception, mem- memory would leave behind some physical imprint, a ory mercenaries slip into a businessman’s dreams to structural, electrical, or biochemical change by which surreptitiously plant an idea. networks of neurons might retain information for hours, Memory-tweaking experiments in the laboratory, months, or years. Researchers collected pieces of this although decidedly more prosaic, are nevertheless puzzle over the years, identifying molecules, genes, yielding dramatic results. In 2013, Susumu Tonegawa and cellular processes that are important for learning at the Massachusetts Institute of Technology in Cam- and memory. Since discoveries in the 1960s and 1970s, bridge, and his colleagues stimulated certain neurons many have suggested that memory is supported by in the hippocampus of mice, demonstrating that the long-term potentiation, an effect by which neurons that researchers could plant a fearful memory of being fire together are able to strengthen their connections. -
From Artificial Intelligence to Brain Intelligence: the Basis Learning and Memory Algorithm for Brain-Like Intelligence Authors: Yifei Mao1*
From Artificial Intelligence to Brain Intelligence: The basis learning and memory algorithm for brain-like intelligence Authors: Yifei Mao1* Affiliations: 1410000 Changsha, Hunan, China. *Corresponding author. Email: [email protected]. Abstract: The algorithm of brain learning and memory is still undetermined. The backpropagation algorithm of artificial neural networks was thought not suitable for brain cortex, and there is a lack of algorithm for memory engram. We designed a brain version of backpropagation algorithm, which are biologically plausible and could be implemented with virtual neurons to complete image classification task. An encoding algorithm that can automatically allocate engram cells is proposed, which is an algorithm implementation for memory engram theory, and could simulate how hippocampus achieve fast associative memory. The role of the LTP and LTD in the cerebellum is also explained in algorithm level. Our results proposed a method for the brain to deploy backpropagation algorithm, and sparse coding method for memory engram theory. Recently, artificial intelligence based on deep neural networks has made great progress in games, medical and other fields (1,2). Backpropagation (BP) as the optimization algorithm of artificial neural network (3), is considered biologically implausible (4). The learning algorithm of biological neural network is still uncertain. The framework Neural Gradient Representation by Activity Differences (NGRAD) was proposed to be a solution for brain to implement BP algorithm, but there is currently a lack of ideal experimental results (5). It is still a mystery that whether the learning algorithm of the cerebral cortex is backpropagation or other algorithms . In terms of memory, The memory engram theory could explain the formation and store of memory in the brain, which was verified by many neuroscience experiments (6,7). -
The Coding Question C.R
TICS 1683 No. of Pages 11 Opinion The Coding Question C.R. Gallistel1,* Recent electrophysiological results imply that the duration of the stimulus Trends onset asynchrony in eyeblink conditioning is encoded by a mechanism intrinsic Recent results suggest that some sim- to the cerebellar Purkinje cell. This raises the general question – how is quanti- ple memories are not stored in plastic tative information (durations, distances, rates, probabilities, amounts, etc.) synapses. transmitted by spike trains and encoded into engrams? The usual assumption The interval between the conditioned is that information is transmitted by firing rates. However, rate codes are stimulus and the unconditioned stimu- energetically inefficient and computationally awkward. A combinatorial code lus in a simple form of Pavlovian con- ditioning appears to be stored in a is more plausible. If the engram consists of altered synaptic conductances (the mechanism intrinsic to the cerebellar usual assumption), then we must ask how numbers may be written to synapses. Purkinje cell. It is much easier to formulate a coding hypothesis if the engram is realized by a How spike trains encode numbers – cell-intrinsic molecular mechanism. the symbols for experienced quantities such as duration, distance, rate, prob- ability, size, and numerosity – is among A Cell-Intrinsic Quantity Memory the most important questions in neuroscience. Recent electrophysiological results imply that individual cerebellar Purkinje cells (see Glossary) time and remember the interval between the onset of an artificial conditioned No code has been proposed for use stimulus (CS) and the onset of an artificial unconditioned stimulus (US) [1] (Figure 1). They with plastic synapses. imply that the timing mechanism is inside the Purkinje cell, as is the engram that encodes the – When it comes to transmission and duration of the CS US interval. -
Memory Engrams: Recalling the Past and Imagining the Future
Memory engrams: Recalling the past and imagining the future The MIT Faculty has made this article openly available. Please share how this access benefits you. Your story matters. Citation Josselyn, Sheena A. and Susumu Tonegawa. "Memory engrams: Recalling the past and imagining the future." Science 367, 6473 (January 2020): eaaw4325 © 2019 The Authors As Published http://dx.doi.org/10.1126/science.aaw4325 Publisher American Association for the Advancement of Science (AAAS) Version Author's final manuscript Citable link https://hdl.handle.net/1721.1/126261 Terms of Use Creative Commons Attribution-Noncommercial-Share Alike Detailed Terms http://creativecommons.org/licenses/by-nc-sa/4.0/ Josselyn & Tonegawa 1 Memory engrams: recalling the past and imaging the future Sheena A. Josselyn1,2,3,4,5 and Susumu Tonegawa6,7 1Program in Neurosciences & Mental Health, Hospital for Sick Children, Toronto, Ontario M5G 1X8, Canada 2Department of Psychology, University of Toronto, Ontario M5S 3G3, Canada 3Department of Physiology, University of Toronto, Ontario M5G 1X8, Canada 4Institute of Medical Sciences, University of Toronto, Ontario M5S 1A8, Canada 5Brain, Mind & Consciousness Program, Canadian Institute for Advanced Research (CIFAR), Toronto, Ontario M5G 1M1, Canada 6RIKEN-MIT Laboratory for Neural Circuit Genetics at the Picower Institute for Learning and Memory, Department of Biology and Department of Brain and Cognitive Sciences, Massachusetts Institute of Technology, Cambridge, MA 02139, U.S.A. 7Howard Hughes Medical Institute, Massachusetts Institute of Technology, Cambridge, MA 02139, U.S.A. Correspondence to: [email protected] or [email protected] Summary Statement Here we review the past, present and future of research examining how information is acquired, stored and used in the brain at the level of the memory engram.