NEO Survey: an Efficient Search for Near-Earth Objects by an IR Observatory in a Venus-Like Orbit
Total Page:16
File Type:pdf, Size:1020Kb
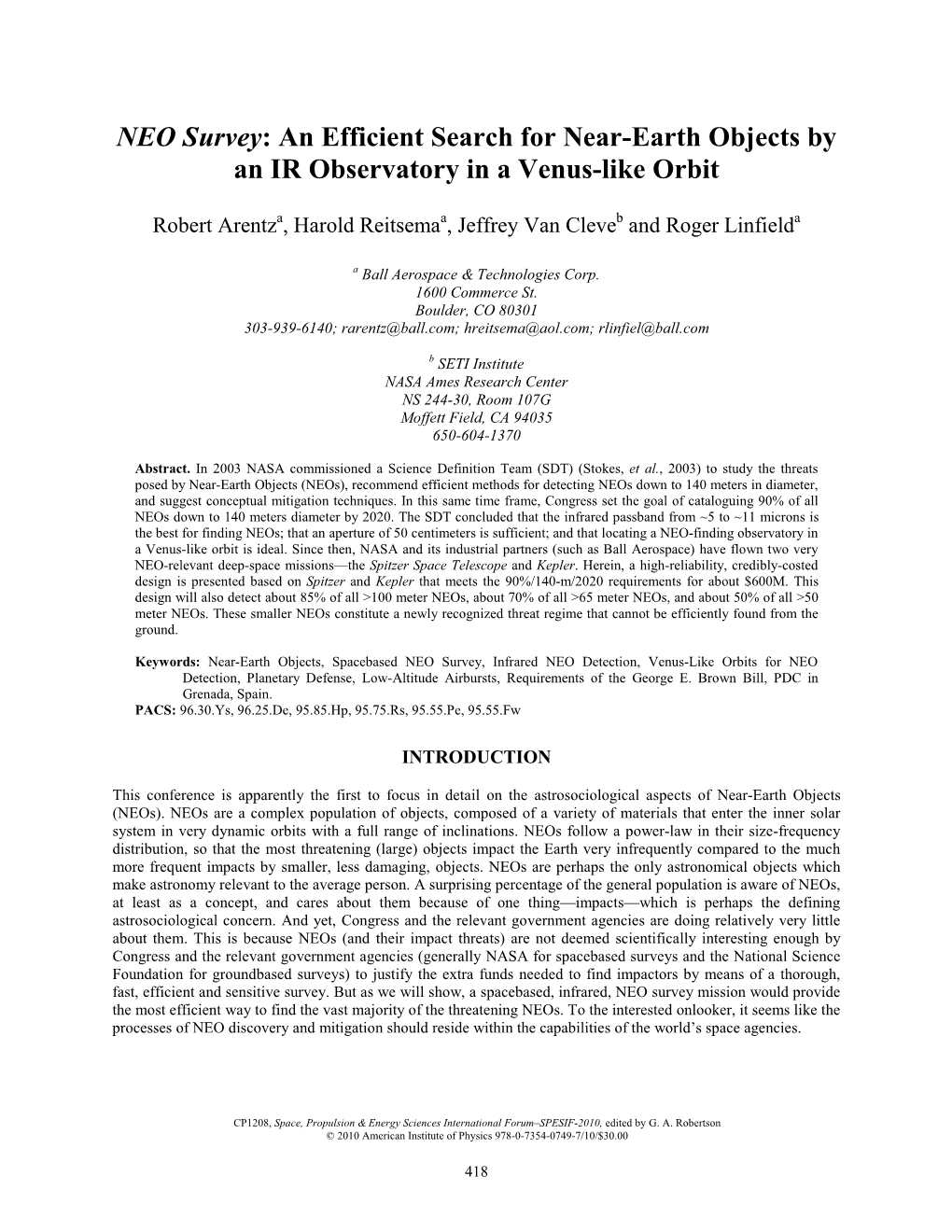
Load more
Recommended publications
-
+ New Horizons
Media Contacts NASA Headquarters Policy/Program Management Dwayne Brown New Horizons Nuclear Safety (202) 358-1726 [email protected] The Johns Hopkins University Mission Management Applied Physics Laboratory Spacecraft Operations Michael Buckley (240) 228-7536 or (443) 778-7536 [email protected] Southwest Research Institute Principal Investigator Institution Maria Martinez (210) 522-3305 [email protected] NASA Kennedy Space Center Launch Operations George Diller (321) 867-2468 [email protected] Lockheed Martin Space Systems Launch Vehicle Julie Andrews (321) 853-1567 [email protected] International Launch Services Launch Vehicle Fran Slimmer (571) 633-7462 [email protected] NEW HORIZONS Table of Contents Media Services Information ................................................................................................ 2 Quick Facts .............................................................................................................................. 3 Pluto at a Glance ...................................................................................................................... 5 Why Pluto and the Kuiper Belt? The Science of New Horizons ............................... 7 NASA’s New Frontiers Program ........................................................................................14 The Spacecraft ........................................................................................................................15 Science Payload ...............................................................................................................16 -
Anticipated Scientific Investigations at the Pluto System
Space Sci Rev (2008) 140: 93–127 DOI 10.1007/s11214-008-9462-9 New Horizons: Anticipated Scientific Investigations at the Pluto System Leslie A. Young · S. Alan Stern · Harold A. Weaver · Fran Bagenal · Richard P. Binzel · Bonnie Buratti · Andrew F. Cheng · Dale Cruikshank · G. Randall Gladstone · William M. Grundy · David P. Hinson · Mihaly Horanyi · Donald E. Jennings · Ivan R. Linscott · David J. McComas · William B. McKinnon · Ralph McNutt · Jeffery M. Moore · Scott Murchie · Catherine B. Olkin · Carolyn C. Porco · Harold Reitsema · Dennis C. Reuter · John R. Spencer · David C. Slater · Darrell Strobel · Michael E. Summers · G. Leonard Tyler Received: 5 January 2007 / Accepted: 28 October 2008 / Published online: 3 December 2008 © Springer Science+Business Media B.V. 2008 L.A. Young () · S.A. Stern · C.B. Olkin · J.R. Spencer Southwest Research Institute, Boulder, CO, USA e-mail: [email protected] H.A. Weaver · A.F. Cheng · R. McNutt · S. Murchie Johns Hopkins University Applied Physics Lab., Laurel, MD, USA F. Bagenal · M. Horanyi University of Colorado, Boulder, CO, USA R.P. Binzel Massachusetts Institute of Technology, Cambridge, MA, USA B. Buratti Jet Propulsion Laboratory, Pasadena, CA, USA D. Cruikshank · J.M. Moore NASA Ames Research Center, Moffett Field, CA, USA G.R. Gladstone · D.J. McComas · D.C. Slater Southwest Research Institute, San Antonio, TX, USA W.M. Grundy Lowell Observatory, Flagstaff, AZ, USA D.P. Hinson · I.R. Linscott · G.L. Tyler Stanford University, Stanford, CA, USA D.E. Jennings · D.C. Reuter NASA Goddard Space Flight Center, Greenbelt, MD, USA 94 L.A. Young et al. -
Newsletter 110 ª June 2002 NEWSLETTER
Newsletter 110 ª June 2002 NEWSLETTER The American Astronomical Societys2000 Florida Avenue, NW, Suite 400sWashington, DC [email protected] AAS NEWS PRESIDENT’S COLUMN Wallerstein is Anneila I. Sargent, Caltech, [email protected] My term as President of the American Astronomical Society Russell Lecturer will end with our meeting in Albuquerque in June 2002. Usually This year, the AAS this letter would be the appropriate place to consider my bestows its highest honor, expectations and goals when I took up the gavel and compare the Henry Norris Russell these with what actually happened. Lectureship on George The events of 11 September 2001 caused me to write that kind Wallerstein, Professor of reflective letter in the December issue of this Newsletter.I Emeritus of Astronomy at won’t repeat myself here except to note that at that time there the University of seemed to be less enthusiasm to fund research in the physical Washington. Wallerstein is sciences than we had grown to expect when I took office. recognized in the award citation for “...his As I write this column, the prospects look much less bleak. In contributions to our another part of this Newsletter, Kevin Marvel discusses how understanding of the astronomy fared in the President’s FY ’03 budget request. George Wallerstein of the University of NASA’s Office of Space Science is doing very well indeed. In Washington will deliver his Russell Lecture abundances of the at the Seattle Meeting in January 2003. elements in stars and fact, the OSS budget has been increasing steadily since 1996 and clusters. -
New Horizons Pluto/KBO Mission Status Report for SBAG
New Horizons Pluto/KBO Mission Status Report for SBAG Hal Weaver NH Project Scientist The Johns Hopkins University Applied Physics Laboratory New Horizons: To Pluto and Beyond KBOs Pluto-Charon Jupiter System 2016-2020 July 2015 Feb-March 2007 Launch Jan 2006 PI: Alan Stern (SwRI) PM: JHU Applied Physics Lab New Horizons is NASA’s first New Frontiers Mission Frontier of Planetary Science Explore a whole new region of the Solar System we didn’t even know existed until the 1990s Pluto is no longer an outlier! Pluto System is prototype of KBOs New Horizons gives the first close-up view of these newly discovered worlds New Horizons Now (overhead view) NH Spacecraft & Instruments 2.1 meters Science Team: PI: Alan Stern Fran Bagenal Rick Binzel Bonnie Buratti Andy Cheng Dale Cruikshank Randy Gladstone Will Grundy Dave Hinson Mihaly Horanyi Don Jennings Ivan Linscott Jeff Moore Dave McComas Bill McKinnon Ralph McNutt Scott Murchie Cathy Olkin Carolyn Porco Harold Reitsema Dennis Reuter Dave Slater John Spencer Darrell Strobel Mike Summers Len Tyler Hal Weaver Leslie Young Pluto System Science Goals Specified by NASA or Added by New Horizons New Horizons Resolution on Pluto (Simulations of MVIC context imaging vs LORRI high-resolution "noodles”) 0.1 km/pix The Best We Can Do Now 0.6 km/pix HST/ACS-PC: 540 km/pix New Horizons Science Status • New Horizons is on track to deliver the goods – The science objectives specified by NASA and the Planetary Community should be achieved, or exceeded • Nix, Hydra, and P4 added (new discoveries) • More data -
Defending Planet Earth: Near-Earth Object Surveys and Hazard Mitigation Strategies Final Report
PREPUBLICATION COPY—SUBJECT TO FURTHER EDITORIAL CORRECTION Defending Planet Earth: Near-Earth Object Surveys and Hazard Mitigation Strategies Final Report Committee to Review Near-Earth Object Surveys and Hazard Mitigation Strategies Space Studies Board Aeronautics and Space Engineering Board Division on Engineering and Physical Sciences THE NATIONAL ACADEMIES PRESS Washington, D.C. www.nap.edu PREPUBLICATION COPY—SUBJECT TO FURTHER EDITORIAL CORRECTION THE NATIONAL ACADEMIES PRESS 500 Fifth Street, N.W. Washington, DC 20001 NOTICE: The project that is the subject of this report was approved by the Governing Board of the National Research Council, whose members are drawn from the councils of the National Academy of Sciences, the National Academy of Engineering, and the Institute of Medicine. The members of the committee responsible for the report were chosen for their special competences and with regard for appropriate balance. This study is based on work supported by the Contract NNH06CE15B between the National Academy of Sciences and the National Aeronautics and Space Administration. Any opinions, findings, conclusions, or recommendations expressed in this publication are those of the author(s) and do not necessarily reflect the views of the agency that provided support for the project. International Standard Book Number-13: 978-0-309-XXXXX-X International Standard Book Number-10: 0-309-XXXXX-X Copies of this report are available free of charge from: Space Studies Board National Research Council 500 Fifth Street, N.W. Washington, DC 20001 Additional copies of this report are available from the National Academies Press, 500 Fifth Street, N.W., Lockbox 285, Washington, DC 20055; (800) 624-6242 or (202) 334-3313 (in the Washington metropolitan area); Internet, http://www.nap.edu. -
Comparing NEO Search Telescopes
Comparing NEO Search Telescopes Nathan Myhrvold Jan 19th, 2016 Abstract Multiple terrestrial and space-based telescopes have been proposed for detecting and tracking near-Earth objects (NEOs). Detailed simulations of the search performance of these systems have used complex computer codes that are not widely available, which hinders accurate cross- comparison of the proposals and obscures whether they have consistent assumptions. Moreover, some proposed instruments would survey infrared (IR) bands, whereas others would operate in the visible band, and differences among asteroid thermal and visible light models used in the simulations further complicate like-to-like comparisons. I use simple physical principles to estimate basic performance metrics for the ground-based Large Synoptic Survey Telescope and three space- based instruments—Sentinel, NEOCam, and a Cubesat constellation. The performance is measured against two different NEO distributions, the Bottke et al. distribution of general NEOs, and the Veres et al. distribution of Earth impacting NEO. The results of the comparison show simplified relative performance metrics, including the expected number of NEOs visible in the search volumes and the initial detection rates expected for each system. Although these simplified comparisons do not capture all of the details, they give considerable insight into the physical factors limiting performance. Multiple asteroid thermal models are considered, including FRM, NEATM, and a new generalized form of FRM (GFRM). I describe issues with how IR albedo and emissivity have been estimated in previous studies, which may render them inaccurate. A thermal model for tumbling asteroids is also developed and suggests that tumbling asteroids may be surprisingly difficult for IR telescopes to observe. -
The Save-The-World Foundation Online
on August 22, 2013 PLANETARY SCIENCE Dodge the bullets. NASA has identifi ed just 1% of the 1 million sizable asteroids thought to swirl close to Earth’s realm. This plot shows the orbits of the The Save-the-World Foundation known Near-Earth Objects more than 140 meters across—those most dangerous should they collide Convinced that NASA will not fi nish the job, a private foundation intends to raise with our planet. www.sciencemag.org $450 million for a space mission to fi nd asteroids that may threaten Earth dation has set its sights on launching a MENLO PARK, CALIFORNIA—In Antoine de a soft spot for Saint-Exupéry and a drive to $450 million mission by July 2018. The infra- Saint-Exupéry’s beloved novella, The Little safeguard fellow citizens have set out to fi nd red telescope, called Sentinel, would spy a Prince, a pilot stranded in the Sahara Desert the rest. half-million NEOs from a vantage point near encounters a being from an asteroid called Planetary scientists agree that a full Venus. B612 has a star-studded team of plan- B-612. “There are also hundreds of others,” inventory of NEOs will require a dedicated etary science veterans and a fi xed-price con- Downloaded from Saint-Exupéry wrote, “some of which are so space observatory—at least a half-billion-dol- tract with Ball Aerospace & Technologies to small that one has a hard time seeing them lar proposition. That’s a stretch for NASA, in build and operate the satellite. through the telescope.” a thin budgetary era when new planetary mis- In the new economy of commercial ven- That figure was far too conserva- sions without “Mars” in the title are rare. -
The B612 Foundation Sentinel Space Telescope
ORIGINAL ARTICLE The B612 Foundation Sentinel Space Telescope Edward T. Lu,1 Harold Reitsema,1 John Troeltzsch,2 NOVEL PRIVATE FUNDING AND COMMERCIAL and Scott Hubbard1,3 PROGRAM MANAGEMENT One of the novel aspects of this mission is the way in which it is 1B612 Foundation, Mountain View, CA. being funded. The B612 Foundation is a nonprofit charitable or- 2Ball Aerospace & Technologies Corp., Boulder, CO. ganization that is raising funds through philanthropic donations. 3Department of Aeronautics and Astronautics, Stanford Interestingly, large ground-based telescopes (such as Lick, Palomar, University, Stanford, CA. Keck, and Yerkes) have historically been mainly funded through philanthropy.4 In some sense, Sentinel will be like these large ob- servatories, with the exception that Sentinel will be in solar orbit ABSTRACT rather than on a mountaintop. The B612 Foundation will, in turn, The B612 Foundation is building, launching, and operating a solar or- contract the spacecraft out to Ball Aerospace and Technologies Corp biting infrared space telescope called Sentinel to find and track aster- (BATC), with B612 functioning in the role of program/contract oids that could impact Earth. Sentinel will be launched in 2018, and manager and carrying out independent assessment of program during the first 6.5 years of operation, it will discover and track the progress. The total cost of the mission is currently under negotia- orbits of more than 90 percent of the population of near-Earth objects tion. The B612 Foundation expects to raise about $450M over the (NEOs) larger than 140 m, and a large fraction of those bigger than the next 12 years to fund all aspects of this mission, including devel- asteroid that struck Tunguska (*45 m). -
Near-Earth Objects
NEAR-EARTH OBJECTS Community White Paper to the Planetary Science Decadal Survey, 2013-2022 Michael Nolan William Merline (Southwest Research Institute) Tel: +1 787 878 2612 extension 212 Patrick Michel (University of Nice-Sophia Antipolis) Arecibo Observatory/Cornell University Beatrice Mueller (Planetary Science Institute) Email: [email protected] Joseph Nuth (NASA Goddard Space Flight Center) David O'Brien (Planetary Science Institute) William Owen (Jet Propulsion Laboratory) Paul Abell (Planetary Science Institute) Joseph Riedel (Jet Propulsion Laboratory) Erik Asphaug (University of California, Santa Cruz) Harold Reitsema (Ball Aerospace, Retired) MiMi Aung (Jet Propulsion Laboratory) Nalin Samarasinha (Planetary Science Institute) Julie Bellerose (JAXA/JSPEC) Daniel Scheeres (University of Colorado) Mehdi Benna (NASA Goddard Space Flight Center) Derek Sears (University of Arkansas) Lance Benner (Jet Propulsion Laboratory) Michael Shepard (Bloomsburg University) David Blewett (Johns Hopkins Applied Physics Lab) Mark Sykes (Planetary Science Institute) William Bottke (Southwest Research Institute) Josep M. Trigo-Rodriguez (Institute of Space Daniel Britt (University of Central Florida) Sciences, CSIC-IEEC) Donald Campbell (Cornell University) David Trilling (Northern Arizona University) Humberto Campins (University of Central Florida) Ronald Vervack (Johns Hopkins Applied Physics Clark Chapman (Southwest Research Institute) Lab) Andrew Cheng (Johns Hopkins Applied Physics Lab) James Walker (Southwest Research Institute) Harold C. Connolly Jr. (Kingsborough Community Benjamin Weiss (Massachusetts Institute of College - CUNY) Technology) Don Davis (Planetary Science Institute) Hajime Yano (JAXA/ISAS & JSPEC) Richard Dissley (Ball Aerospace) Donald Yeomans (Jet Propulsion Laboratory) Gerhard Drolshagen (ESA/ESTEC) Eliot Young (Southwest Research institute) Dan Durda (Southwest Research Institute) Michael Zolensky (NASA Johnson Space Center) Eugene Fahnestock (Jet Propulsion Laboratory) Yanga Fernandez (University of Central Florida) Michael J. -
Private Asteroid Hunt Lacks Cash
NEWS IN FOCUS the light, which strongly suggested that it date would also explain why the stars were Until now, many astronomers believed that came from the first generation of stars. visible to Subaru. seeing the first generation of stars would take CR7 also hosts second-generation stars, That primordial stars should turn up in a an instrument such as NASA’s James Webb made from material spat out by dying first- large and evolved galaxy presents a challenge Space Telescope, which is projected to cost generation stars, which means that it is not the to the group’s interpretation of CR7’s light nearly US$9 billion, is scheduled to launch sort of galaxy in which astronomers had imag signal, but is probably the least exotic of the in 2018 and should have the power to look ined that they would make such a discovery. possible explanations, says Naoki Yoshida, an further back in time than any previous instru Sobral and his colleagues suggest that the pri astrophysicist at the University of Tokyo. One ment. But if Sobral and his colleagues are right, mordial stars may be late developers, formed alternative, that the emission comes from a it may be possible to see primordial stars with from a cloud of pristine and uncontaminated supermassive black hole that formed directly existing telescopes. Indeed, similar bright gal gas that was prevented from cooling and coa from the collapse of a pristine cloud of gas, axies have already been identified as potential lescing by the heat of strong radiation from would actually be “even more spectacular”, candidates, adds Sobral. -
Protecting the Earth from Asteroid Impacts
PROTECTING THE EARTH FROM ASTEROID IMPACTS ANNUAL PROGRESS REPORT 2018 1 It is time for space agencies, private corporations, academics, and nonprofits to work together with the goal of filling in the details on the millions of asteroids in our inner solar system. DANICA REMY AND ED LU, “SPACE MINING, ASTEROIDS, AND WHY WE NEED TO MAP THE INNER SOLAR SYSTEM,” FINANCIAL TIMES Cover: South African Coast, by Ed Lu from the ISS This page: Manicouagan Impact Crater is located in central Quebec and is believed to have been created approximately 214 (±1) million years ago by an asteroid impact roughly 5 km in size. By Ed Lu, from the ISS LETTER FROM THE PRESIDENT Over the last six years at B612, we’ve seen our work grow and This summer, the U.S. government updated its plan for asteroid impact awareness and evolve thanks to the support from our donors. We have built prevention. We applaud NASA’s articulation of the steps to implement the National Near- alliances and partnerships with groups in the field and the Earth Object Preparedness Strategy and Action Plan, and we see a continuing need to private sector and have co-founded Asteroid Day, a worldwide support the development of new telescopes for discovering and tracking asteroids and movement focused on increasing asteroid education. We are the means for calculating and understanding their orbits. advancing technologies that show new promise in finding In addition to advancing the technology to support the field, we continue to advocate and tracking asteroids, and we are building powerful cloud- and educate about the value of developing a comprehensive dynamic map of the inner based tools to analyze the asteroid data from telescopes around the world. -
New Horizons: Anticipated Scientific Investigations at the Pluto System
Sp. Sci. Rev. New Horizons: Anticipated Scientific Investigations Young et al. New Horizons: Anticipated Scientific Investigations at the Pluto System Leslie A. Young1*, S. Alan Stern1, Harold A. Weaver2, Fran Bagenal3, Richard P. Binzel4, Bonnie Buratti5, Andrew F. Cheng2, Dale Cruikshank6, G. Randall Gladstone7, William M. Grundy8, David P. Hinson9, Mihaly Horanyi3, Donald E. Jennings10, Ivan R. Linscott9, David J. McComas7, William B. McKinnon11, Ralph McNutt2, Jeffery M. Moore6, Scott Murchie2, Carolyn C. Porco12, Harold Reitsema13, Dennis C. Reuter10, John R. Spencer1, David C. Slater7, Darrell Strobel14, Michael E. Summers15, G. Leonard Tyler9 1Southwest Research Institute, Boulder CO, U.S.A. 2Johns Hopkins University Applied Physics Lab., Laurel MD, U.S.A. 3University of Colorado, Boulder CO, U.S.A. 4Massachusetts Institute of Technology, Cambridge MA, U.S.A. 5Jet Propulsion Laboratory, Pasadena CA, U.S.A. 6NASA Ames Research Center, Moffett Field CA, U.S.A. 7Southwest Research Institute, San Antonio TX, U.S.A. 8Lowell Observatory, Flagstaff AZ, U.S.A. 9Stanford University, Stanford CA, U.S.A. 10NASA Goddard Space Flight Center, Greenbelt MD, U.S.A. 11Washington University, Saint Louis MO, U.S.A. 12Space Science Institute, Boulder CO, U.S.A. 13Ball Aerospace and Technologies Corporation, Boulder CO, U.S.A. 14Johns Hopkins University, Baltimore MD 15George Mason University, Fairfax, VA, U.S.A. (*Author for correspondence: email [email protected]) Submitted to Space Science Review Jan 2, 2007: 1 Sp. Sci. Rev. New Horizons: Anticipated Scientific Investigations Young et al. Abstract The New Horizons spacecraft will achieve a wide range of measurement objectives at the Pluto system, including color and panchromatic maps, 1.25-2.50 micron spectral images for studying surface compositions, and measurements of Pluto's atmosphere (temperatures, composition, hazes, and the escape rate).