Flagellar Perturbations Activate Adhesion Through Two Distinct Pathways in Caulobacter Crescentus
Total Page:16
File Type:pdf, Size:1020Kb
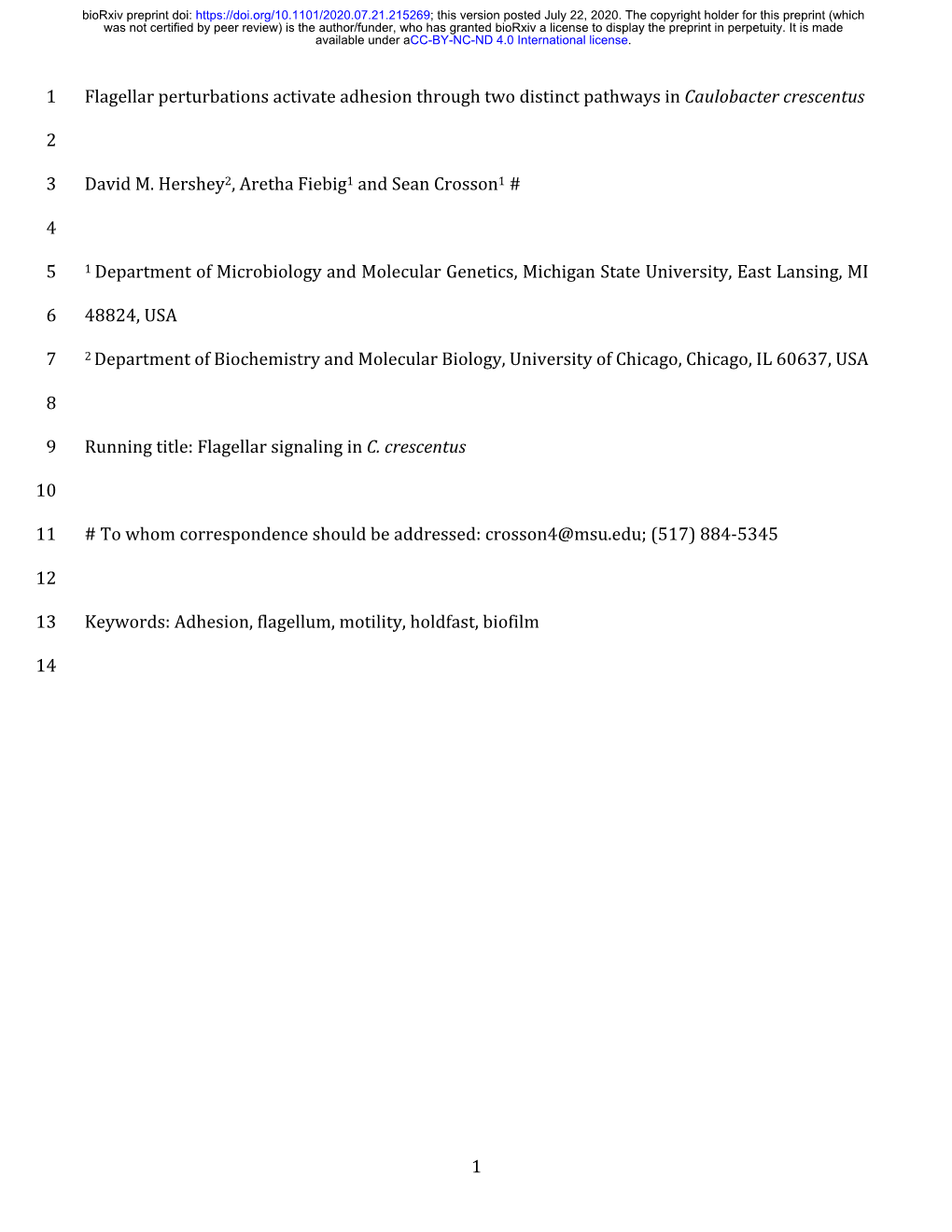
Load more
Recommended publications
-
Caulobacter Crescentus
Biochemistry of the key spatial regulators MipZ and PopZ in Caulobacter crescentus Dissertation zur Erlangung des Doktorgrades der Naturwissenschaften (Dr. rer. nat.) dem Fachbereich Biologie der Philipps-Universität Marburg vorgelegt von Yacine Refes aus Algier, Algerien Marburg, im November 2017 Vom Fachbereich Biologie der Philipps-Universität Marburg (Hochschulkennziffer: 1180) als Dissertation angenommen am: 22.01.2018 Erstgutachter: Prof. Dr. Martin Thanbichler Zweitgutachter: Prof. Dr. Torsten Waldminghaus Tag der mündlichen Prüfung am: 26.01.2018 Die Untersuchungen zur vorliegenden Arbeit wurden von November 2013 bis Juni 2017 am Max-Planck-Institut für terrestrische Mikrobiologie und an der Philipps Universität Marburg unter der Leitung von Prof. Dr. Martin Thanbichler durchgeführt. Publications Refes Y, He B, Corrales-Guerrero L, Steinchen W, Bange G, and Thanbichler M. Determinants of DNA binding by the bacterial cell division regulator MipZ. In preparation Corrales-Guerrero L, Refes Y, He B, Ramm B, Muecksch J, Steinchen W, Heimerl T, Knopp J, Bange G, Schwille P, and Thanbichler M. Regulation of cell division protein FtsZ by MipZ in Caulobacter crescentus. In preparation ...dans les champs de l'observation le hasard ne favorise que les esprits préparés - Louis Pasteur Abstract Bacteria are known to tightly control the spatial distribution of certain proteins by positioning them to distinct regions of the cell, particularly the cell poles. These regions represent important organizing platforms for several processes essential for bacterial survival and reproduction. The proteins localized at the cell poles are recruited to these positions by interaction with other polar proteins or protein complexes. The α-proteobacterium Caulobacter crescentus possesses a self- organizing polymeric polar matrix constituted of the scaffolding protein PopZ. -
Regulation of Cell Fate Asymmetry in Caulobacter Crescentus by a Complex of Two Component Signaling Proteins
Regulation of Cell Fate Asymmetry in Caulobacter crescentus by a Complex of Two Component Signaling Proteins Christos G. Tsokos AUG 0 3 201 B.A. Biological Sciences Li0RA R ISF Cornell University, Ithaca, NY, 2004 ARCHIVES SUBMITTED TO THE DEPARTMENT OF BIOLOGY IN PARTIAL FULFILLMENT OF THE REQUIREMENTS FOR THE DEGREE OF DOCTOR OF PHILOSOPHY IN BIOLOGY AT THE MASSACHUSETTS INSTITUTE OF TECHNOLOGY SEPTEMBER 2011 0 2011 Christos G. Tsokos. All rights reserved. The author hereby grants MIT permission to reproduce and distribute publicly paper and electronic copies of this thesis document in whole or in part. Signature of Author: _________G._______ Christos G. Tsokos Department of Biology June 14, 2011 Certified by: Michael T. Laub Associate Professor of Biology Thesis supervisor Accepted by: Alan D. Grossman Praecis Professor of Biology Regulation of Cell Fate Asymmetry in Caulobacter crescentus by a Complex of Two Component Signaling Proteins by Christos G. Tsokos Submitted to the Department of Biology on June 14, 2011 in partial fulfillment of the requirements for the degree of Doctor of Philosophy in Biology at the Massachusetts Institute of Technology Abstract Cellular asymmetry is critical to the generation of complexity in both metazoans and many microbes. However, several molecular mechanisms responsible for translating asymmetry into differential cell fates remain unknown. Caulobacter crescentus provides an excellent model to study this process because every division is asymmetric. One daughter cell, the stalked cell, is sessile and commits immediately to S phase. The other daughter, the swarmer cell, is motile and locked in G1. Cellular differentiation requires asymmetric distribution or activation of regulatory factors. -
The Role of Caulobacter Cell Surface Structures in Colonization of the Air-Liquid Interface
bioRxiv preprint doi: https://doi.org/10.1101/524058; this version posted April 14, 2019. The copyright holder for this preprint (which was not certified by peer review) is the author/funder, who has granted bioRxiv a license to display the preprint in perpetuity. It is made available under aCC-BY-NC 4.0 International license. THE ROLE OF CAULOBACTER CELL SURFACE STRUCTURES IN COLONIZATION OF THE AIR-LIQUID INTERFACE ARETHA FIEBIG Department of Biochemistry and Molecular Biology, The University of Chicago, Chicago, IL [email protected] Abstract In aquatic environments, Caulobacter spp. are often present at the boundary between liquid and air known as the neuston. I report an approach to study temporal features of Caulobacter crescentus colonization and pellicle biofilm development at the air-liquid interface, and have defined the role of cell surface structures in this process. At this interface, C. crescentus initially forms a monolayer of cells bearing a surface adhesin known as the holdfast. When excised from the liquid surface, this monolayer strongly adheres to glass. The monolayer subsequently develops into a three-dimensional structure that is highly enriched in clusters of stalked cells known as rosettes. As this pellicle film matures, it becomes more cohesive and less adherent to a glass surface. A mutant strain lacking a flagellum does not efficiently reach the surface, and strains lacking type IV pili exhibit defects in organization of the three-dimensional pellicle. Strains unable to synthesize holdfast fail to accumulate at the boundary between air and liquid and do not form a pellicle. Phase contrast images support a model whereby the holdfast functions to trap C. -
A Novel and Conserved Dnaa-Related Protein That Targets The
bioRxiv preprint doi: https://doi.org/10.1101/712307; this version posted July 23, 2019. The copyright holder for this preprint (which was not certified by peer review) is the author/funder. All rights reserved. No reuse allowed without permission. HdaB: a novel and conserved DnaA-related protein that targets the RIDA process to stimulate replication initiation Antonio Frandi and Justine Collier* Department of Fundamental Microbiology, Faculty of Biology and Medicine, University of Lausanne, Quartier UNIL/Sorge, Lausanne, CH 1015, Switzerland *Correspondance: E-mail: [email protected] Telephone: +41 21 692 5610 Fax: +41 21 692 5605 1 bioRxiv preprint doi: https://doi.org/10.1101/712307; this version posted July 23, 2019. The copyright holder for this preprint (which was not certified by peer review) is the author/funder. All rights reserved. No reuse allowed without permission. ABSTRACT Exquisite control of the DnaA initiator is critical to ensure that bacteria initiate chromosome replication in a cell cycle-coordinated manner. In many bacteria, the DnaA-related and replisome-associated Hda/HdaA protein interacts with DnaA to trigger the regulatory inactivation of DnaA (RIDA) and prevent over-initiation events. In the C. crescentus Alphaproteobacterium, the RIDA process also targets DnaA for its rapid proteolysis by Lon. The impact of the RIDA process on adaptation of bacteria to changing environments remains unexplored. Here, we identify a novel and conserved DnaA-related protein, named HdaB, and show that homologs from three different Alphaproteobacteria can inhibit the RIDA process, leading to over-initiation and cell death when expressed in actively growing C. crescentus cells. -
The Development of Cellular Stalks in Bacteria
View metadata, citation and similar papers at core.ac.uk brought to you by CORE provided by PubMed Central THE DEVELOPMENT OF CELLULAR STALKS IN BACTERIA JEAN M. SCHMIDT and R. Y. STANIER From the Department of Bacteriology and Immunology and the Electron Microscope Labora- tory, University of California, Berkeley ABSTRACT Extensive stalk elongation in Caulobacter and Asticcacaulis can bc obtained in a dcfincd medium by limiting thc concentration of phosphate. Caulobacter cells which wcre initiating stalk formation werc labeled with tritiatcd glucose. After rcmoval of exogenous tritiated material, the cells wcrc sul~jected to phosphate limitation whilc stalk elongation occurred. Thc location of tritiatcd material in the elongated stalks as dctected by radioautographic techniques allowed identification of the site of stalk development. Thc labeling pattern ob- tained was consistent with the hypothesis that the materials of the stalk are synthcsizcd at thc juncturc of the stalk with the cell. Complemcntary labcling experiments with Caulo- bacter and Asticcacaulis confirmed this result. In spheroplasts of C. crescentus preparcd by treatment with lysozyme, the stalks lost their normal rigid outline after several minutes of cxposurc to the enzyme, indicating that the rigid layer of the cell wall attacked by lysozyme is present in the stalk. In spheroplasts of growing cells induced with penicillin, the stalks did not appear to be affected, indicating that the stalk wall is a relatively inert, nongrowing structure. The morphogcnetic implications of these findings are discussed. INTRODUCTION The family Caulobacteraceae consists of rod- characteristically secreted at the pole of the cell. shaped or vibrioid bacteria, which can produce In Caulobacter, the holdfast consequently occurs filiform extensions of the cell, devoid of reproduc- around the terminal end of the stalk, but in tive function, known as stalks. -
Coping with Stress
Coping with Stress Regulation of the Caulobacter crescentus cell cycle in response to environmental cues Kristina Heinrich Academic dissertation for the Degree of Doctor of Philosophy in Molecular Bioscience at Stockholm University to be publicly defended on Friday 21 September 2018 at 10.00 in Vivi Täckholmsalen, NPQ-huset, Svante Arrhenius väg 20 A. Abstract All organisms have to respond to environmental changes to maintain cellular and genome integrity. In particular, unicellular organisms like bacteria must be able to analyze their surroundings and rapidly adjust their growth mode and cell cycle program in response to environmental changes, such as changes in nutrient availability, temperature, osmolarity, or pH. Additionally, they have to compete with other species for nutrients and evade possible predators or the immune system. Bacteria exhibit a myriad of sophisticated regulatory pathways that allow them to cope with various kinds of threats and ensure their survival. However, the precise molecular mechanisms underlying these responses remain in many cases incompletely described. This thesis focuses on the mechanisms that adjust growth and cell cycle progression of Caulobacter crescentus under adverse conditions. In paper I we describe a mechanism by which environmental information is transduced via the membrane-bound cell cycle kinase CckA into the cell division program of C. crescentus. This mechanism ensures rapid dephosphorylation and clearance of the cell cycle master regulator CtrA under salt and ethanol stress. The downregulation of CtrA leads to a cell division block and cell filamentation, which provides a growth advantage under these conditions. Cell filamentation of C. crescentus can also be observed in the late stationary phase, in which a small subpopulation of cells transforms into helical shaped filaments. -
The Regulation of Dnaa in Caulobacter Crescentus
The Regulation of DnaA in Caulobacter crescentus Richard Burns Wargachuk Department of Microbiology and Immunology McGill University, Montreal August 2012 A thesis submitted to McGill University in partial fulfillment of the requirements of the degree of Doctor of Philosophy © Richard Burns Wargachuk, 2012 Table of Contents Table of Contents ............................................................................................................................. I Table of Figures .............................................................................................................................. IV Abstract ......................................................................................................................................... VI Résumé .......................................................................................................................................... IX Acknowledgements ....................................................................................................................... XII Contributions to original knowledge ........................................................................................... XIII Chapter One ................................................................................................................................... 1 Overview of the literature review ............................................................................................... 2 Prokaryotic Chromosome Replication .................................................................................... -
Replication Initiator Dnaa Binds at the Caulobacter Centromere and Enables Chromosome Segregation
Replication initiator DnaA binds at the Caulobacter centromere and enables chromosome segregation Paola E. Mera, Virginia S. Kalogeraki, and Lucy Shapiro1 Department of Developmental Biology, Beckman Center, Stanford University School of Medicine, Palo Alto, CA 94305 Contributed by Lucy Shapiro, October 1, 2014 (sent for review September 5, 2014; reviewed by James W. Gober and Matthew K. Waldor) During cell division, multiple processes are highly coordinated to number of ori (Fig. 1A). In a wild-type background, initiation of faithfully generate genetically equivalent daughter cells. In bacte- DNA replication results in the migration of one copy of the CFP- ria, the mechanisms that underlie the coordination of chromosome ParB/parS complex toward the opposite end of the cell, culmi- replication and segregation are poorly understood. Here, we nating in the establishment of a second fluorescent ParB focus at report that the conserved replication initiator, DnaA, can mediate the distal pole (7, 11) (Fig. 1 A and B). To investigate the de- chromosome segregation independent of replication initiation. It pendency of chromosome segregation on DNA replication, we does so by binding directly to the parS centromere region of the imaged the translocation of CFP-ParB/parS foci in cells expressing chromosome, and mutations that alter this interaction result in subphysiological DnaA concentrations. We constructed a Caulobacter cells that display aberrant centromere translocation and cell divi- strain in which transcription of the sole copy of dnaA was controlled sion. We propose that DnaA serves to coordinate bacterial DNA by the tightly regulated, xylose-inducible PxylX promoter (15) and in parB replication with the onset of chromosome segregation. -
Regulation of Brucella Abortus Catalase As a Defensive Mechanism Against Oxidative Stress Jeonga Kim Iowa State University
Iowa State University Capstones, Theses and Retrospective Theses and Dissertations Dissertations 1997 Regulation of Brucella abortus catalase as a defensive mechanism against oxidative stress Jeonga Kim Iowa State University Follow this and additional works at: https://lib.dr.iastate.edu/rtd Part of the Immunology and Infectious Disease Commons, Medical Immunology Commons, Microbiology Commons, and the Molecular Biology Commons Recommended Citation Kim, Jeonga, "Regulation of Brucella abortus catalase as a defensive mechanism against oxidative stress " (1997). Retrospective Theses and Dissertations. 12219. https://lib.dr.iastate.edu/rtd/12219 This Dissertation is brought to you for free and open access by the Iowa State University Capstones, Theses and Dissertations at Iowa State University Digital Repository. It has been accepted for inclusion in Retrospective Theses and Dissertations by an authorized administrator of Iowa State University Digital Repository. For more information, please contact [email protected]. INFORMATION TO USERS This manuscript has been reproduced fi-om the microfihn master. UMI fihns the text directly from the original or copy submitted. Thus, some thesis and dissertation copies are in typewriter &ce, \^e others may be from any type of computer printer. The quality of this reproduction is dependent upon the quality of the copy submitted. Broken or indistinct print, colored or poor quality illustrations and photographs, print bleedthrough, substandard margins, and improper alignment can adversely affect reproduction. In the unlikely event that the author did not send UMI a complete manuscript and there are missing pages, these will be noted. Also, if unauthorized copyright material had to be removed, a note will indicate the deletion. -
Development in Caulobacter Crescentus (Dlfferentiation/Phosphorylation/Cell Cyde) SHUI PING WANG, PREM L
Proc. Nati. Acad. Sci. USA Vol. 90, pp. 630-634, January 1993 Developmental Biology A histidine protein kinase is involved in polar organelle development in Caulobacter crescentus (dlfferentiation/phosphorylation/cell cyde) SHUI PING WANG, PREM L. SHARMA*, PATRICIA V. SCHOENLEINt, AND BERT ELYt Department of Biological Sciences, University of South Carolina, Columbia, SC 29208 Communicated by Richard M. Losick, September 3, 1992 ABSTRACT Mutations having pleiotropic effects on polar the flagellin proteins, are nonchemotactic, and have a re- organelle development (pod) in Caulobacter crescentus have duced ability to release assembled flagallar filaments prior to been identified and shown to occur in at least 13 genes scattered stalk development (1, 2). Also, pleC mutants are resistant to throughout the genome. Mutations at each locus affect a unique polar bacteriophage, produce a paralyzed flagellum, and are combination of polar traits, suggesting that complex interac- stalkless in the presence of excess phosphate (3-5). In tions occur among these genes. The DNA sequence of one of contrast, pleA mutants are resistant to polar bacteriophage these genes, pkeC, indites that it is homologous to members and do not synthesize a flagellum (3-5). It has been suggested of the family of histidine protein kinase genes. Members ofthis that these genes encode regulatory elements that control the family include the sensor components of the bacterial two- expression of genes involved in polar development (1, 2, 5). component regulatory systems. Furthermore, in vitro experi- To provide evidence that these genes encode regulatory ments demonstrated that the PleC protein was capable of elements, we have cloned, sequenced, and analyzed one of autophosphorylation. -
The Caulobacter Crescentus Flagellar Gene, Flix, Encodes a Novel Trans-Acting Factor That Couples Flagellar Assembly to Transcription
Molecular Microbiology (2001) 39(6), 1623±1637 The Caulobacter crescentus flagellar gene, fliX, encodes a novel trans-acting factor that couples flagellar assembly to transcription Rachel E. Muir, Tina M. O'Brien and James W. Gober* Introduction Department of Chemistry and Biochemistry and the, The dimorphic bacterium, Caulobacter crescentus, under- Molecular Biology Institute, University of California, goes an asymmetric division every cell cycle, resulting in Los Angeles, CA 90095-1569, USA. the formation of dissimilar progeny cells: a motile, flagellum-bearing swarmer cell and a sessile stalked Summary cell. The biogenesis of the polar flagellum is the best- studied aspect of this programme of cell differentiation The first flagellar assembly checkpoint of Caulobacter (reviewed in Brun et al., 1994; Gober and Marques, 1995; crescentus couples assembly of the early class II Wu and Newton, 1997; Gober and England, 2000). The components of the basal body complex to the temporal synthesis of the flagellum is subject to a complex expression of class III and IV genes, which encode regulatory hierarchy that functions to co-ordinate the extracytoplasmic structures of the flagellum. The expression of approximately 50 flagellar genes with transcription of class III/IV flagellar genes is activated progression through the cell cycle and the developmental by the response regulator factor, FlbD. Gain of function state of the flagellum. Caulbacter crescentus flagellar mutations in flbD,termedbfa, can bypass the tran- genes have been grouped, according to epistasis data, scriptional requirement for the assembly of class II into a regulatory hierarchy of four classes (Bryan et al., flagellar structures. Here we show that the class II 1984; Ohta et al., 1985, 1991; Champer et al., 1987; flagellar gene fliX encodes a trans-acting factor that Minnich and Newton, 1987; Newton et al., 1989; Xu et al., couples flagellar assembly to FlbD-dependent tran- 1989; Ramakrishnan et al., 1994; Mangan et al., 1995). -
G1-Arrested Newborn Cells Are the Predominant Infectious Form of the Pathogen Brucella Abortus
ARTICLE Received 25 Oct 2013 | Accepted 10 Jun 2014 | Published 9 Jul 2014 DOI: 10.1038/ncomms5366 OPEN G1-arrested newborn cells are the predominant infectious form of the pathogen Brucella abortus Michae¨l Deghelt1,*, Caroline Mullier1,*, Jean- Franc¸ois Sternon1, Nayla Francis1,Ge´raldine Laloux2,3,4, Delphine Dotreppe1, Charles Van der Henst1, Christine Jacobs-Wagner2,3,4, Jean-Jacques Letesson1 & Xavier De Bolle1 Several intracellular pathogens, such as Brucella abortus, display a biphasic infection process starting with a non-proliferative stage of unclear nature. Here, we study the cell cycle of B. abortus at the single-cell level, in culture and during infection of HeLa cells and macrophages. The localization of segregation and replication loci of the two bacterial chromosomes indicates that, immediately after being engulfed by host-cell endocytic vacuoles, most bacterial cells are newborn. These bacterial cells do not initiate DNA replication for the next 4 to 6 h, indicating a G1 arrest. Moreover, growth is completely stopped during that time, reflecting a global cell cycle block. Growth and DNA replication resume later, although bacteria still reside within endosomal-like compartments. We hypothesize that the predominance of G1-arrested bacteria in the infectious population, and the bacterial cell cycle arrest following internalization, may constitute a widespread strategy among intracellular pathogens to colonize new proliferation niches. 1 Microorganisms biology research unit (URBM), University of Namur (UNamur), Namur, Belgium. 2 Department of Molecular, Cellular and Developmental Biology, Yale University, New Haven, Connecticut 06520, USA. 3 Howard Hughes Medical Institute, Yale University, New Haven, Connecticut 06520, USA. 4 Department of Microbial Pathogenesis, Yale School of Medicine, New Haven, Connecticut 06510, USA.