Structural and Functional Roles of Nebulin in Skeletal Muscle
Total Page:16
File Type:pdf, Size:1020Kb
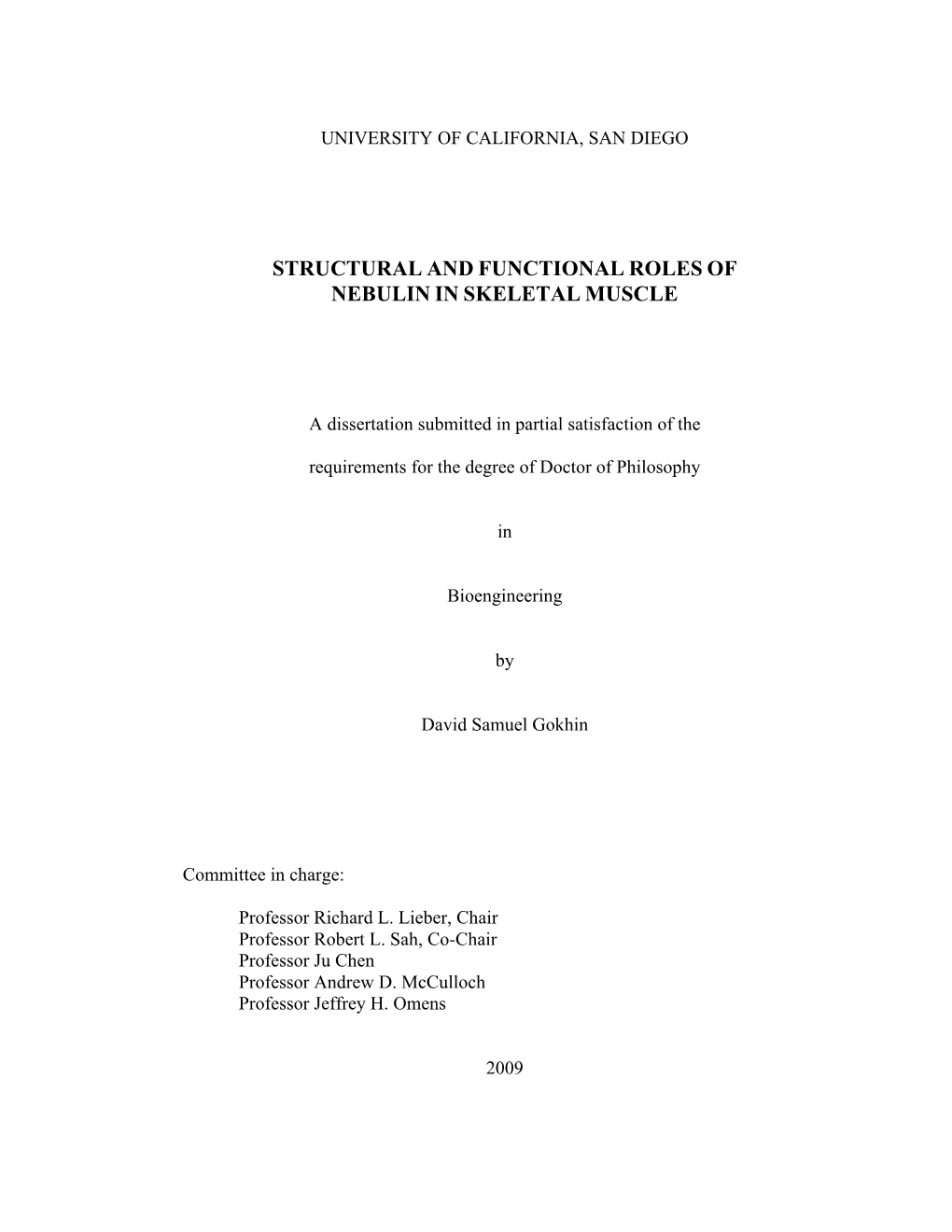
Load more
Recommended publications
-
The Role of Z-Disc Proteins in Myopathy and Cardiomyopathy
International Journal of Molecular Sciences Review The Role of Z-disc Proteins in Myopathy and Cardiomyopathy Kirsty Wadmore 1,†, Amar J. Azad 1,† and Katja Gehmlich 1,2,* 1 Institute of Cardiovascular Sciences, College of Medical and Dental Sciences, University of Birmingham, Birmingham B15 2TT, UK; [email protected] (K.W.); [email protected] (A.J.A.) 2 Division of Cardiovascular Medicine, Radcliffe Department of Medicine and British Heart Foundation Centre of Research Excellence Oxford, University of Oxford, Oxford OX3 9DU, UK * Correspondence: [email protected]; Tel.: +44-121-414-8259 † These authors contributed equally. Abstract: The Z-disc acts as a protein-rich structure to tether thin filament in the contractile units, the sarcomeres, of striated muscle cells. Proteins found in the Z-disc are integral for maintaining the architecture of the sarcomere. They also enable it to function as a (bio-mechanical) signalling hub. Numerous proteins interact in the Z-disc to facilitate force transduction and intracellular signalling in both cardiac and skeletal muscle. This review will focus on six key Z-disc proteins: α-actinin 2, filamin C, myopalladin, myotilin, telethonin and Z-disc alternatively spliced PDZ-motif (ZASP), which have all been linked to myopathies and cardiomyopathies. We will summarise pathogenic variants identified in the six genes coding for these proteins and look at their involvement in myopathy and cardiomyopathy. Listing the Minor Allele Frequency (MAF) of these variants in the Genome Aggregation Database (GnomAD) version 3.1 will help to critically re-evaluate pathogenicity based on variant frequency in normal population cohorts. -
Structural Biology of the Dystrophin-Deficient Muscle Fiber
Dystrophin-deficient muscle fiber REVIEW ISSN- 0102-9010145 STRUCTURAL BIOLOGY OF THE DYSTROPHIN-DEFICIENT MUSCLE FIBER Maria Julia Marques Department of Anatomy, Institute of Biology, State University of Campinas (UNICAMP), Campinas, SP, Brazil. ABSTRACT The discovery of dystrophin and its gene has led to major advances in our understanding of the molecular basis of Duchenne, Becker and other muscular dystrophies related to the dystrophin-associated protein complex. The concept that dystrophin has a mechanical function in stabilizing the muscle fiber membrane has expanded in the last five years. The dystrophin-glycoprotein complex is now considered a multifunctional complex that contains molecules involved in signal transduction cascades important for cell survival. The roles of dystrophin and the dystrophin- glycoprotein complex in positioning and anchoring receptors and ion channels is also important, and much of what is known about these functions is based on studies of the neuromuscular synapse. In this review, we discuss the components and the cellular signaling molecules associated with the dystrophin-glycoprotein complex. We then focus on the molecular organization of the neuromuscular junction and its structural organization in the dystrophin-deficient muscle fibers of mdx mice, a well-established experimental model of Duchenne muscular dystrophy. Key words: Confocal microscopy, Duchenne muscular dystrophy, mdx, neuromuscular junction INTRODUCTION that can improve the quality of life of the patients, Duchenne muscular dystrophy (DMD) is an X- death usually occurs in the early twenties, as a result linked recessive, progressive muscle-wasting disease of cardiac and/or respiratory failures [24]. that affects primarily skeletal and cardiac muscle. Several other muscular dystrophies have been DMD was first reported in 1868 by Dr. -
Influence of Nebulin on the Assembly Mechanics Of
INFLUENCE OF NEBULIN ON THE ASSEMBLY MECHANICS OF DESMIN An Undergraduate Research Scholars Thesis by MARC ADRIAN CARAGEA Submitted to Honors and Undergraduate Research Texas A&M University In partial fulfillment of the requirements for the designation as UNDERGRADUATE RESEARCH SCHOLAR Approved by Research Adviser: Gloria M. Conover, Ph.D. May 2014 Major: Biomedical Sciences TABLE OF CONTENTS Page ABSTRACT ............................................................................................................................... 1 ACKNOWLEDGMENTS ........................................................................................................... 2 NOMENCLATURE .................................................................................................................... 3 CHAPTER I INTRODUCTION ............................................................................................... 4 II MATERIALS AND METHODS ......................................................................... 8 Affinity purification of WT and mutant desmin proteins from bacteria ................ 8 Affinity purification of recombinant Nebulin M160 – 164 ................................... 9 Assembly protocol for desmin precursors into filaments in vitro ...................... 11 Sample preparation for atomic force microscopy ............................................... 11 Single desmin filament length acquisition and analysis ..................................... 13 Determination of Young’s modulus for desmin filament networks ................... -
Troponin Variants in Congenital Myopathies: How They Affect Skeletal Muscle Mechanics
International Journal of Molecular Sciences Review Troponin Variants in Congenital Myopathies: How They Affect Skeletal Muscle Mechanics Martijn van de Locht , Tamara C. Borsboom, Josine M. Winter and Coen A. C. Ottenheijm * Department of Physiology, Amsterdam Cardiovascular Sciences, Amsterdam UMC, Location VUmc, 1081 HZ Amsterdam, The Netherlands; [email protected] (M.v.d.L.); [email protected] (T.C.B.); [email protected] (J.M.W.) * Correspondence: [email protected]; Tel.: +31-(0)-20-444-8123 Abstract: The troponin complex is a key regulator of muscle contraction. Multiple variants in skeletal troponin encoding genes result in congenital myopathies. TNNC2 has been implicated in a novel congenital myopathy, TNNI2 and TNNT3 in distal arthrogryposis (DA), and TNNT1 and TNNT3 in nemaline myopathy (NEM). Variants in skeletal troponin encoding genes compromise sarcomere function, e.g., by altering the Ca2+ sensitivity of force or by inducing atrophy. Several potential therapeutic strategies are available to counter the effects of variants, such as troponin activators, introduction of wild-type protein through AAV gene therapy, and myosin modulation to improve muscle contraction. The mechanisms underlying the pathophysiological effects of the variants in skeletal troponin encoding genes are incompletely understood. Furthermore, limited knowledge is available on the structure of skeletal troponin. This review focusses on the physiology of slow and fast skeletal troponin and the pathophysiology of reported variants in skeletal troponin encoding genes. A better understanding of the pathophysiological effects of these variants, together with enhanced knowledge regarding the structure of slow and fast skeletal troponin, will direct the development of Citation: van de Locht, M.; treatment strategies. -
Distal Myopathies a Review: Highlights on Distal Myopathies with Rimmed Vacuoles
Review Article Distal myopathies a review: Highlights on distal myopathies with rimmed vacuoles May Christine V. Malicdan, Ikuya Nonaka Department of Neuromuscular Research, National Institutes of Neurosciences, National Center of Neurology and Psychiatry, Tokyo, Japan Distal myopathies are a group of heterogeneous disorders Since the discovery of the gene loci for a number classiÞ ed into one broad category due to the presentation of distal myopathies, several diseases previously of weakness involving the distal skeletal muscles. The categorized as different disorders have now proven to recent years have witnessed increasing efforts to identify be the same or allelic disorders (e.g. distal myopathy the causative genes for distal myopathies. The identiÞ cation with rimmed vacuoles and hereditary inclusion body of few causative genes made the broad classiÞ cation of myopathy, Miyoshi myopathy and limb-girdle muscular these diseases under “distal myopathies” disputable and dystrophy type 2B (LGMD 2B). added some enigma to why distal muscles are preferentially This review will focus on the most commonly affected. Nevertheless, with the clariÞ cation of the molecular known and distinct distal myopathies, using a simple basis of speciÞ c conditions, additional clues have been classification: distal myopathies with known molecular uncovered to understand the mechanism of each condition. defects [Table 1] and distal myopathies with unknown This review will give a synopsis of the common distal causative genes [Table 2]. The identification of the myopathies, presenting salient facts regarding the clinical, genes involved in distal myopathies has broadened pathological, and molecular aspects of each disease. Distal this classification into sub-categories as to the location myopathy with rimmed vacuoles, or Nonaka myopathy, will of encoded proteins: sarcomere (titin, myosin); plasma be discussed in more detail. -
Effect of Nebulin Variants on Nebulin-Actin Interaction
Pro gradu –thesis EFFECT OF NEBULIN VARIANTS ON NEBULIN-ACTIN INTERACTION Svetlana Sofieva November 2019 University of Helsinki Genetics Folkhälsan research center Specialization in Human Genetics Tiedekunta – Fakultet – Faculty Laitos – Institution– Department Faculty of biological and environmental sciences Department of Biosciences Tekijä – Författare – Author Svetlana Sofieva Työn nimi – Arbetets titel – Title Effect of nebulin variants on nebulin-actin interaction Oppiaine – Läroämne – Subject Human genetics Työn laji – Arbetets art – Level Aika – Datum – Month and year Sivumäärä – Sidoantal – Number of pages Master’s thesis 11/2019 61 pages + 15 pages in Appendix Tiivistelmä – Referat – Abstract Nemaline myopathy (NM) is a rare congenital disorder, the most common of congenital myopathies. It affects primarily the skeletal muscles and it is recognised by nemaline bodies in muscle tissue samples and muscle weakness. Mutation of eleven genes are known to lead to NM and the most frequent disease-causing variants are either recessive NEB variants or dominant ACTA1 variants. Variants in NEB are thought to be well tolerated and only 7% of them are hypothesized to be pathogenic. Over 200 pathogenic NEB- variants have been identified in Helsinki and the majority occurred in patients as a combination of two different variants. The missense variants were speculated to have a modifying effect on pathogenicity by affecting nebulin-actin or nebulin-tropomyosin interactions. Nebulin is a gigantic protein coded by NEB and is one of the largest proteins in vertebrates. It is located in the thin filament of the skeletal muscle sarcomere. Enclosed by terminal regions, nebulin has an extensive repetitive modular region that covers over 90% of the protein. -
Diagnosis and Cell-Based Therapy for Duchenne Muscular Dystrophy in Humans, Mice, and Zebrafish
J Hum Genet (2006) 51:397–406 DOI 10.1007/s10038-006-0374-9 MINIREVIEW Louis M. Kunkel Æ Estanislao Bachrach Richard R. Bennett Æ Jeffrey Guyon Æ Leta Steffen Diagnosis and cell-based therapy for Duchenne muscular dystrophy in humans, mice, and zebrafish Received: 3 January 2006 / Accepted: 4 January 2006 / Published online: 1 April 2006 Ó The Japan Society of Human Genetics and Springer-Verlag 2006 Abstract The muscular dystrophies are a heterogeneous mutants carries a stop codon mutation in dystrophin, group of genetically caused muscle degenerative disor- and we have recently identified another carrying a ders. The Kunkel laboratory has had a longstanding mutation in titin. We are currently positionally cloning research program into the pathogenesis and treatment of the disease-causative mutation in the remaining 12 mu- these diseases. Starting with our identification of dys- tant strains. We hope that one of these new mutant trophin as the defective protein in Duchenne muscular strains of fish will have a mutation in a gene not previ- dystrophy (DMD), we have continued our work on ously implicated in human muscular dystrophy. This normal dystrophin function and how it is altered in gene would become a candidate gene to be analyzed in muscular dystrophy. Our work has led to the identifi- patients which do not carry a mutation in any of the cation of the defective genes in three forms of limb girdle known dystrophy-associated genes. By studying both muscular dystrophy (LGMD) and a better understand- disease pathology and investigating potential therapies, ing of how muscle degenerates in many of the different we hope to make a positive difference in the lives of dystrophies. -
Typology and Profile of Spine Muscles. Structure of Myofibrils and Role of Protein Components – Review of Current Literature
Ovidius University Annals, Series Physical Education and Sport / SCIENCE, MOVEMENT AND HEALTH Vol. XI, ISSUE 2 Supplement, 2011, Romania The JOURNAL is nationally acknowledged by C.N.C.S.I.S., being included in the B+ category publications, 2008-2011. The journal is indexed in: Ebsco, SPORTDiscus, INDEX COPERNICUS JOURNAL MASTER LIST, DOAJ DIRECTORY OF OPEN ACCES JOURNALS, Caby, Gale Cengace Learning TYPOLOGY AND PROFILE OF SPINE MUSCLES. STRUCTURE OF MYOFIBRILS AND ROLE OF PROTEIN COMPONENTS – REVIEW OF CURRENT LITERATURE STRATON ALEXANDRU1, ENE-VOICULESCU CARMEN1, GIDU DIANA1, PETRESCU ANDREI1 Abstract. Muscular profile of spine muscles has a great importance in trunk stability. It seems that muscles which support the spine show a high content of red muscle fiber with a cross section area equal or higher than white muscle fibers. It is possible that lumbar extensor muscles to have different functional capacity between sexes. Most of the myofibril structural proteins except protein actin and protein myosin have a role in maintaining the structural integrity of muscle cell. Key words: muscle, fibres, myofibrils, proteins, spine. thoracic muscle structure lying superficial and deep is Introduction composed of 74% type I fibers, lumbar muscle Proper understanding of muscle fibers profile of structure located superficially is composed of 57% muscles supporting the spine and the role of muscle type I fibers and lumbar muscle structure located deep cell structural proteins leads to better achievements in is composed of 63% type I fibers. Type I muscle fiber performance training and rehabilitation. diameter is significantly larger than that of type II fibers. Another study, conducted on 42 patients Muscle fibers typology divided into two groups - 21 patients with lumbar Skeletal muscle contains two major types of back pain and 21 patients without lumbar back pain muscle fibers: slow twitch red or type I fibers) and almost identical groups as gender, age and body mass fast twitch white or type II fibers). -
Postmortem Changes in the Myofibrillar and Other Cytoskeletal Proteins in Muscle
BIOCHEMISTRY - IMPACT ON MEAT TENDERNESS Postmortem Changes in the Myofibrillar and Other C'oskeletal Proteins in Muscle RICHARD M. ROBSON*, ELISABETH HUFF-LONERGAN', FREDERICK C. PARRISH, JR., CHIUNG-YING HO, MARVIN H. STROMER, TED W. HUIATT, ROBERT M. BELLIN and SUZANNE W. SERNETT introduction filaments (titin), and integral Z-line region (a-actinin, Cap Z), as well as proteins of the intermediate filaments (desmin, The cytoskeleton of "typical" vertebrate cells contains paranemin, and synemin), Z-line periphery (filamin) and three protein filament systems, namely the -7-nm diameter costameres underlying the cell membrane (filamin, actin-containing microfilaments, the -1 0-nm diameter in- dystrophin, talin, and vinculin) are listed along with an esti- termediate filaments (IFs), and the -23-nm diameter tubu- mate of their abundance, approximate molecular weights, lin-containing microtubules (Robson, 1989, 1995; Robson and number of subunits per molecule. Because the myofibrils et al., 1991 ).The contractile myofibrils, which are by far the are the overwhelming components of the skeletal muscle cell major components of developed skeletal muscle cells and cytoskeleton, the approximate percentages of the cytoskel- are responsible for most of the desirable qualities of muscle eton listed for the myofibrillar proteins (e.g., myosin, actin, foods (Robson et al., 1981,1984, 1991 1, can be considered tropomyosin, a-actinin, etc.) also would represent their ap- the highly expanded corollary of the microfilament system proximate percentages of total myofibrillar protein. of non-muscle cells. The myofibrils, IFs, cell membrane skel- eton (complex protein-lattice subjacent to the sarcolemma), Some Important Characteristics, Possible and attachment sites connecting these elements will be con- Roles, and Postmortem Changes of Key sidered as comprising the muscle cell cytoskeleton in this Cytoskeletal Proteins review. -
Calmodulin-Binding Profiles for Nebulin and Dystrophin in Human Skeletal Muscle
View metadata, citation and similar papers at core.ac.uk brought to you by CORE provided by Elsevier - Publisher Connector Volume 234, number 2, 267-271 FEB 06034 July 1988 Calmodulin-binding profiles for nebulin and dystrophin in human skeletal muscle K. Patel, P.N. Strong, V. Dubowitz and M.J. Dunn Jerry Lewis Muscle Research Centre, Department of Paediairics and Neonatal Medicine, Royal Postgraduate Medical School, London WI2 ONN, EngIand Received 20 April 1988; revised version received 15 May 1988 Nebulin and dystrophin are two high-molecular-mass skeletal muscle proteins that have both been associated with the defective gene in Duchenne muscular dystrophy, although the function of neither protein is known. Other high-molecu- lar-mass, calmodulin-binding proteins have recently been implicated in regulating calcium release from skeletal muscle. Western blots of human skeletal muscle biopsy samples were probed with biotinylated calmodulin; nebulin was identified as a prominent high-molecular-mass calmodulin-binding protein but dystrophin did not bind detectable amounts of bio- tinylated calmodulin. Dystrophin was absent in a Duchenne muscle biopsy. Muscle protein; Duchenne muscular dystrophy; Nebulin; Dystrophin; Calmodulin; SDS-PAGE 1. INTRODUCTION with the defective gene product in Duchenne muscular dystrophy 131, has recently been shown The structure and function of high-molecular- to fractionate with junctional feet membrane mass proteins in junctional sarcoplasmic reticulum preparations, although it is distinct (both im- and the -
Skeletal Muscle in Aged Mice Reveals Extensive Transformation of Muscle
Lin et al. BMC Genetics (2018) 19:55 https://doi.org/10.1186/s12863-018-0660-5 RESEARCHARTICLE Open Access Skeletal muscle in aged mice reveals extensive transformation of muscle gene expression I-Hsuan Lin1†, Junn-Liang Chang3†, Kate Hua1, Wan-Chen Huang4, Ming-Ta Hsu2 and Yi-Fan Chen4* Abstract Background: Aging leads to decreased skeletal muscle function in mammals and is associated with a progressive loss of muscle mass, quality and strength. Age-related muscle loss (sarcopenia) is an important health problem associated with the aged population. Results: We investigated the alteration of genome-wide transcription in mouse skeletal muscle tissue (rectus femoris muscle) during aging using a high-throughput sequencing technique. Analysis revealed significant transcriptional changes between skeletal muscles of mice at 3 (young group) and 24 (old group) months of age. Specifically, genes associated with energy metabolism, cell proliferation, muscle myosin isoforms, as well as immune functions were found to be altered. We observed several interesting gene expression changes in the elderly, many of which have not been reported before. Conclusions: Those data expand our understanding of the various compensatory mechanisms that can occur with age, and further will assist in the development of methods to prevent and attenuate adverse outcomes of aging. Keywords: Aging, Skeletal muscle, Cardiac-related genes, RNA sequencing analysis, Muscle fibers, Defects on differentiation Background SIRT1 reduces the oxidative stress and inflammation Aging is a process whereby various changes were accu- associated with ameliorating diseases, such as vascular mulated over time, resulting in dysfunction in mole- endothelial disorders, neurodegenerative diseases, as cules, cells, tissues and organs. -
The Role of Nuclear Positioning in Muscle Function
UNIVERSIDADE DE LISBOA Faculdade de Medicina The role of nuclear positioning in muscle function Mafalda Ramos de Melo Pimentel Orientador: Prof. Doutor Edgar Rodrigues Almeida Gomes Tese especialmente elaborada para obtenção do grau de Doutor em Ciências Biomédicas especialidade em Biologia Celular e Molecular 2019 UNIVERSIDADE DE LISBOA Faculdade de Medicina The role of nuclear positioning in muscle function Mafalda Ramos de Melo Pimentel Orientador: Prof. Doutor Edgar Rodrigues Almeida Gomes Tese especialmente elaborada para obtenção do grau de Doutor em Ciências Biomédicas especialidade em Biologia Celular e Molecular Júri: Presidente: Doutor João Eurico Cortez Cabral da Fonseca, Professor Catedrático e Vice-Presidente do Conselho Cientifico da Faculdade de Medicina da Universidade de Lisboa Vogais: Doctor Antoine Guichet, Group Leader and Principal Investigator, Institut Jacques Monod, Université Paris Diderot; Doutor Reto Gassmann, Group Leader and Investigador do Instituto de Biologia Molecular e Celular da Universidade do Porto; Doutor Ramiro Daniel Carvalho de Almeida, Professor Auxiliar do Departamento de Ciências Médicas da Universidade de Aveiro; Doutora Solveig Thorsteinsdottir, Professora Associada com Agregação da Faculdade de Ciências da Universidade de Lisboa; Doutora Maria do Carmo Salazar Velez Roque da Fonseca, Professora Catedrática da Faculdade de Medicina da Universidade de Lisboa; Doutor Edgar Rodrigues Almeida Gomes, Professor Associado Convidado da Faculdade de Medicina da Universidade de Lisboa; Instituição Financiadora: Fundação para a Ciência e Tecnologia SFRH/BD/52227/2013 2019 A impressão desta tese foi aprovada pelo Conselho Científico da Faculdade de Medicina de Lisboa em reunião de 16 de Outubro de 2018. As opiniões expressas nesta publicação são da exclusiva respondabilidade do seu autor.