The Origin of Asian Monsoons: a Modelling Perspective
Total Page:16
File Type:pdf, Size:1020Kb
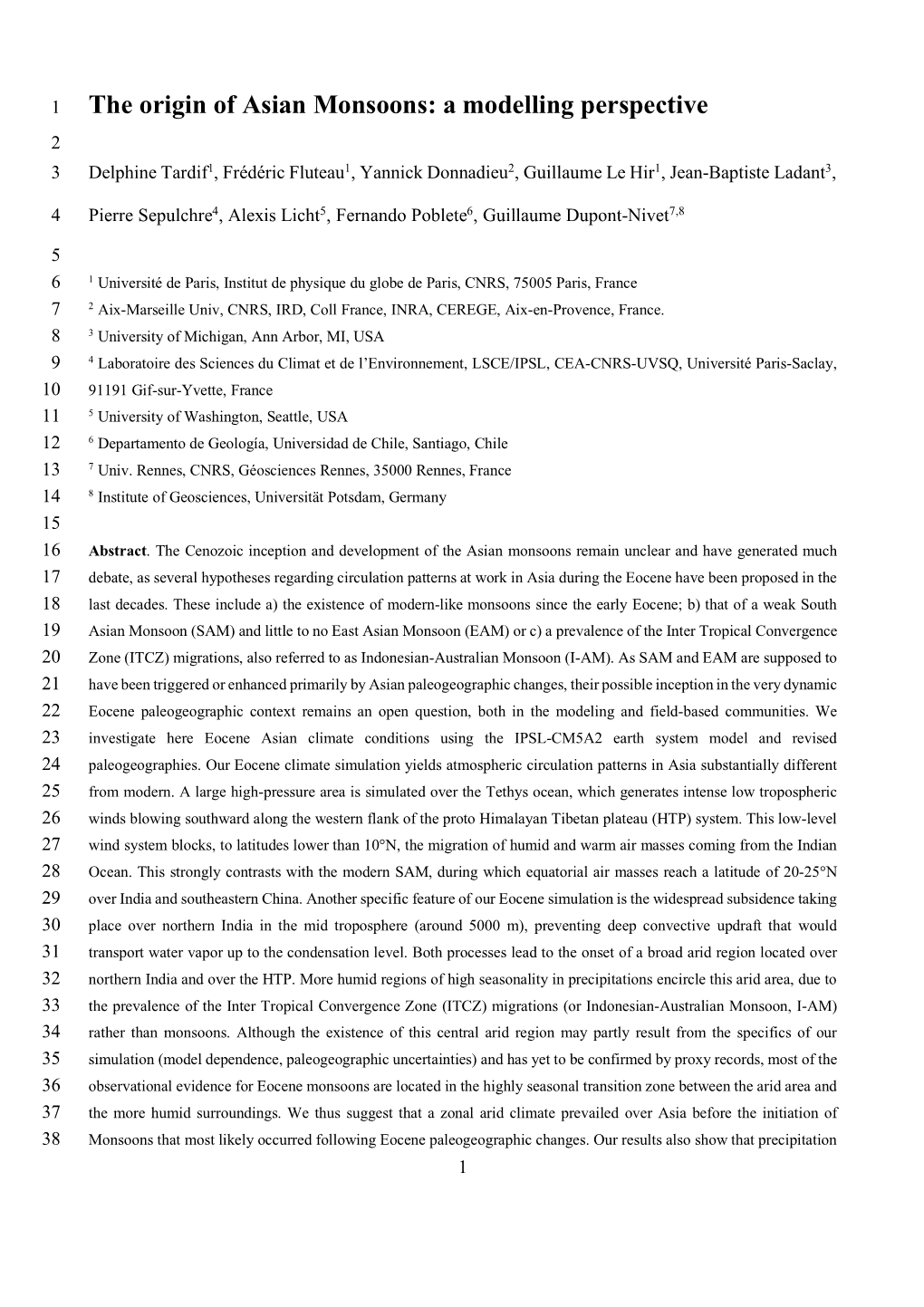
Load more
Recommended publications
-
Review of Human-Elephant FINAL Reduced 01.Cdr
Prithiviraj Fernando, M. Ananda Kumar, A. Christy Williams, Eric Wikramanayake, Tariq Aziz, Sameer M. Singh WORLD BANK-WWF ALLIANCE FOR FOREST CONSERVATION & SUSTAINABLE USE Review of Human-Elephant Conflict Mitigation Measures Practiced in South Asia (AREAS Technical Support Document Submitted to World Bank) Prithiviraj Fernando, M. Ananda Kumar, A. Christy Williams, Eric Wikramanayake, Tariq Aziz, Sameer M. Singh Published in 2008 by WWF - World Wide Fund for Nature. Any reproduction in full or in part of this publication must mention the title and credit the above mentioned publisher as the copyright owner. © text and graphics: 2008 WWF. All rights reserved. Photographs by authors as credited. CONTENTS Preamble 1-2 LIST OF TECHNIQUES Problem Animal Removal 28-33 Traditional Crop Protection 3-7 Capture and domestication Capture and semi-wild management Crop guarding Elimination Noise and Throwing Things Fire Compensation & Insurance 34-35 Supplements to traditional crop protection Land-Use Planning 36-38 Alarms Providing benefits from conservation to Repellants Local communities Organized Crop Protection 8-11 Recommendations 39 Guard teams, 40-43 Vehicle patrols, References Cited Koonkies Literature Cited 44-45 Elephant Barriers 12-18 Physical FORMAT FOR Wire fences EACH TECHNIQUE Log and stone fences Technique Ditches Applicable scale Biological fences Objective Psychological Description of technique Electric fences Positive effects Cleared boundaries and simple demarcation of fields People Elephants Buffer Crops & Unpalatable Crops 19-20 Negative effects People Supplementary Feeding 21-22 Elephants Translocation 23-27 Future needs Chemical immobilization and transport In-country applications Elephant drives Sri Lanka PREAMBLE ew wild species evoke as much attention and varied emotions from humans as elephants. -
The Global Monsoon Across Time Scales Mechanisms And
Earth-Science Reviews 174 (2017) 84–121 Contents lists available at ScienceDirect Earth-Science Reviews journal homepage: www.elsevier.com/locate/earscirev The global monsoon across time scales: Mechanisms and outstanding issues MARK ⁎ ⁎ Pin Xian Wanga, , Bin Wangb,c, , Hai Chengd,e, John Fasullof, ZhengTang Guog, Thorsten Kieferh, ZhengYu Liui,j a State Key Laboratory of Mar. Geol., Tongji University, Shanghai 200092, China b Department of Atmospheric Sciences, School of Ocean and Earth Science and Technology, University of Hawaii at Manoa, Honolulu, HI 96825, USA c Earth System Modeling Center, Nanjing University of Information Science and Technology, Nanjing 210044, China d Institute of Global Environmental Change, Xi'an Jiaotong University, Xi'an 710049, China e Department of Earth Sciences, University of Minnesota, Minneapolis, MN 55455, USA f CAS/NCAR, National Center for Atmospheric Research, 3090 Center Green Dr., Boulder, CO 80301, USA g Key Laboratory of Cenozoic Geology and Environment, Institute of Geology and Geophysics, Chinese Academy of Sciences, P.O. Box 9825, Beijing 100029, China h Future Earth, Global Hub Paris, 4 Place Jussieu, UPMC-CNRS, 75005 Paris, France i Laboratory Climate, Ocean and Atmospheric Studies, School of Physics, Peking University, Beijing 100871, China j Center for Climatic Research, University of Wisconsin Madison, Madison, WI 53706, USA ARTICLE INFO ABSTRACT Keywords: The present paper addresses driving mechanisms of global monsoon (GM) variability and outstanding issues in Monsoon GM science. This is the second synthesis of the PAGES GM Working Group following the first synthesis “The Climate variability Global Monsoon across Time Scales: coherent variability of regional monsoons” published in 2014 (Climate of Monsoon mechanism the Past, 10, 2007–2052). -
High-Latitude Climate Zones and Climate Types - E.I
ENVIRONMENTAL STRUCTURE AND FUNCTION: CLIMATE SYSTEM – Vol. II - High-Latitude Climate Zones and Climate Types - E.I. Khlebnikova HIGH-LATITUDE CLIMATE ZONES AND CLIMATE TYPES E.I. Khlebnikova Main Geophysical Observatory, St.Petersburg, Russia Keywords: annual temperature range, Arctic continental climate, Arctic oceanic climate, katabatic wind, radiation cooling, subarctic continental climate, temperature inversion Contents 1. Introduction 2. Climate types of subarctic and subantarctic belts 2.1. Continental climate 2.2. Oceanic climate 3. Climate types in Arctic and Antarctic Regions 3.1. Climates of Arctic Region 3.2. Climates of Antarctic continent 3.2.1. Highland continental region 3.2.2. Glacial slope 3.2.3. Coastal region Glossary Bibliography Biographical Sketch Summary The description of the high-latitude climate zone and types is given according to the genetic classification of B.P. Alisov (see Genetic Classifications of Earth’s Climate). In dependence on air mass, which is in prevalence in different seasons, Arctic (Antarctic) and subarctic (subantarctic) belts are distinguished in these latitudes. Two kinds of climates are considered: continental and oceanic. Examples of typical temperature and precipitation regime and other meteorological elements are presented. 1. IntroductionUNESCO – EOLSS In the high latitudes of each hemisphere two climatic belts are distinguished: subarctic (subantarctic) andSAMPLE arctic (antarctic). CHAPTERS The regions with the prevalence of arctic (antarctic) air mass in winter, and polar air mass in summer, belong to the subarctic (subantarctic) belt. As a result of the peculiarities in distribution of continents and oceans in the northern hemisphere, two types of climate are distinguished in this belt: continental and oceanic. In the southern hemisphere there is only one type - oceanic. -
Climate of South and Southeast Asia According to Thornthwaite's Classification Scheme Author(S)
View metadata, citation and similar papers at core.ac.uk brought to you by CORE provided by Kyoto University Research Information Repository <Notes>Climate of South and Southeast Asia according to Title Thornthwaite's Classification Scheme Author(s) Kyuma, Kazutake Citation 東南アジア研究 (1971), 9(1): 136-158 Issue Date 1971-06 URL http://hdl.handle.net/2433/55650 Right Type Journal Article Textversion publisher Kyoto University Tonan Ajia Kenkyu (The Southeast Asian Studies) Vol. 9, No. 1 June, 1971 Climate of South and Southeast Asia according to Thornthwaite's Classification Scheme by Kazutake KYUMA* Introduction In the course of the study of the paddy soils in South and Southeast Asia (Project Leader: Prof. K. Kawaguchi, Faculty of Agriculture, Kyoto University) there are many instances where soil distribution is governed primarily by climate. The occurrence of Grumusols in a region that has a distinctly dry season is an oft-quoted examples of this sort. One of the most striking examples of differing soil distribution governed by climate is seen in Ceylon. Irrespective of the similarity of parent rocks underlying a greater part of the island, Reddish Brown Earths are the dominant soils in the dry zone as against associations of Red-Yellow Podzolic Soils and Red-Yellow Latosols in the wet zone. Thus, in attempting to acquire a better understanding of soil forming conditions over the entire paddy-growing area of tropical and subtropical Asia, climate must first be made the subject of a detailed study. There are several schemes for classifying world climates, the one proposed by Koppen being the most well known. -
USGS Open-File Report 2010-1099
25th Himalaya-Karakoram-Tibet Workshop San Francisco – June 2010 The Climate of Asia and Tibet – Not Just a Simple Monsoon Gerard H. Roe1 1 Department of Earth and Space Sciences, University of Washington, Seattle, WA 98195, USA, [email protected] Asia, the largest continuous landmass, experiences the greatest seasonality and climate gradients on Earth, and induces an atmospheric circulation that each year draws more than half the air mass of a hemisphere across the equator. Tibet, the largest topographic feature outside of the poles, sits square in the middle of the jet stream and induces atmospheric circulation patterns that affect climate around the globe. Within Earth Sciences, and also many textbooks, the climate of Asia is often deconstructed into two semi- annual components: a wintertime, dry cold monsoon, associated with the Siberian high-pressure system; and a summertime, warm wet monsoon associated with a thermal low-pressure system over the continent. In the extreme of this cartoon, the whole Indian-Asian monsoon system is depicted as a single dynamical system, driven by the thermal contrast between land and ocean. In actuality, this vast tract of real estate experiences a rich spatial structure of atmospheric circulation and related weather. In many cases these varied phenomena have largely independent causes. Moreover some of the most interesting features of the climate dynamics defy a simple deconstruction into two monsoonal seasons. Some examples of this: in fall, northern Asia experiences the greatest circulation -
Ss21 Wcgeog Text.Pdf
Exploring World Cultural Geography INSTRUCTIONS Welcome to your Continental Academy course “Exploring Worl d Cultural Geography”. It is made up of 8 indivi dual lessons, as listed in the Table of Contents. Each lesson includes practice questions with answers. You will progress through this course one lesson at a time, at your own pace. First, study the lesson thoroughly. Then, complete the lesson reviews at the end of the lesson and carefully check your answers. Sometimes, those answers will contain information t hat you will need on the graded lesson assignments. When you are ready, complete the 10-question, multiple choice lesson assignment. At the end of each lesson, you will find notes t o help you prepare for the online assignments. All lesson assignments are open-book. Continue working on the lessons at your own pace until you have finished all lesson assignments for this course. When you have completed and passed all lesson assignments for this course, complete the End of Course Examination. If you need help understanding any part of the lesson, practice questions, or this procedure: . Click on the “Send a Message” link on the left side of the home page . Select “Academic Guidance” in the “To” field . Type your question in the field provided . Then, click on the “Send” button . You will receive a response within ONE BUSINESS DAY 2 Exploring World Cultural Geography About the Author… Caroline Grant earned her Bachelor of Arts [B.A.] degree in Psychology and her Master of Science [M.S.] Degree in Social Studies Education from Florida International University. -
General Rainfall Patterns in Indonesia and the Potential Impacts of Local Seas on Rainfall Intensity
Water 2015, 7, 1751-1768; doi:10.3390/w7041751 OPEN ACCESS water ISSN 2073-4441 www.mdpi.com/journal/water Article General Rainfall Patterns in Indonesia and the Potential Impacts of Local Seas on Rainfall Intensity Han Soo Lee 1,2 1 Graduate School of Science and Engineering, Saitama University, 255 Shimo-Okubo, Sakura-Ku, Saitama 338-8570, Japan; E-Mail: [email protected]; Tel./Fax: +81-48-858-3571 2 International Institute for Resilient Society, Saitama University, 255 Shimo-Okubo, Sakura-Ku, Saitama 338-8570, Japan Academic Editor: Maria Filomena Camões Received: 30 December 2014 / Accepted: 14 April 2015 / Published: 22 April 2015 Abstract: The relationships between observed rainfall, El Niño/Southern Oscillation (ENSO) and sea surface temperature (SST) variations in the Pacific and Indian Oceans were analyzed using a 1° latitude–longitude grid over Indonesia. The Global Summary of the Day rainfall records provide 26 years of rainfall data (January 1985 to August 2010) for 23 stations throughout the Indonesian islands. The ENSO and SST variations were calculated using the Multivariate ENSO Index (MEI), the Pacific Decadal Oscillation (PDO), NINO1 + 2, NINO3, NINO3.4, NINO4, the Dipole Mode Index (DMI) for the Indian Ocean Dipole (IOD), and Indian Ocean Basin-wide (IOBW) index. The results show that the rainfall in the southern Sumatra and southern Java Islands, which face the Indian Ocean, was positively correlated with the negative IOD, whereas the rainfall in northwestern Sumatra was positively correlated with the positive IOD. In eastern Indonesia, the rainfall was positively correlated with La Niña. The PDO index was also strongly correlated with the rainfall in this region. -
A Report 2019
CLIMATE SMART DISASTER RISK REDUCTION INTERVENTIONS IN AGRICULTURE SECTOR – FLOOD HAZARD A Report 2019 CLIMATE SMART DISASTER RISK REDUCTION INTERVENTIONS IN AGRICULTURE SECTOR – FLOOD HAZARD A Report 2019 This publication may be freely quoted but acknowledgement of the source is requested. © ADPC 2019. Citation Basnayake, S, Punyawardena, BVR, Jayasinghe, S, Gupta, N, Shrestha, ML and Premalal, KHMS 2019. Climate Smart Disaster Risk Reduction Interventions in Agriculture Sector – Flood hazard – A Report, Asian Disaster Preparedness Center. The publication is an output of the project titled “Developing Climate Inclusive Potential Loss and Damage Assessment Methodology for Flood Hazards” implemented by Asian Disaster Preparedness Center in collaboration with Deakin University, Australia, Department of Meteorology, Government of Sri Lanka and The Small Earth Nepal. The project was funded by the Asia-Pacific Network for Global Change Research, Japan under their Collaborative Regional Research Programme (APN Reference: CAF2016-RR01-CMY-Basnayake). Contributors from partner countries: Thailand: Dr. Senaka Basnayake, Mr. Susantha Jayasinghe, Dr. Niladri Gupta Sri Lanka: Mr. KHMS Premalal, Mr. DA Jayasinghearachchi Nepal: Dr. Madan Lall Shrestha, Mr. Dilli Bhattarai, Mr. AP Bhattarai, Mr. Nammy Hang Kirat Australia: Dr. Mehmet Ali Ulubaşoğlu, Dr. Muhammad Habibur Rahman, Mr. Cahit Güven Cover Photo Courtesy: Reuters/Sukree Sukplang and IRRI Executive Summary Climate Change is inevitable and the agriculture and water sectors are the most vulnerable. Recent studies have shown that due to climate change, the world is moving towards scenarios of either too much, or too little, water. Agriculture is an open system and provides livelihoods for 60% of the world’s population. More than 2.2 billion people depend on agriculture for their livelihoods in Asia. -
Aerosol and Monsoon Climate Interactions Over Asia 10.1002/2015RG000500 Zhanqing Li1,2, W
PUBLICATIONS Reviews of Geophysics REVIEW ARTICLE Aerosol and monsoon climate interactions over Asia 10.1002/2015RG000500 Zhanqing Li1,2, W. K.-M. Lau2, V. Ramanathan3,G.Wu4, Y. Ding5, M. G. Manoj2, J. Liu2, Y. Qian6,J.Li1, 4 6 7 8 9 10 11,12 13 2 Key Points: T. Zhou , J. Fan , D. Rosenfeld , Y. Ming , Y. Wang , J. Huang , B. Wang ,X.Xu , S.-S. Lee , 2 1 1 1 14 1 4 12 5 13 • The fast-developing Asia has suffered M. Cribb , F. Zhang , X. Yang , C. Zhao , T. Takemura , K. Wang , X. Xia , Y. Yin , H. Zhang , J. Guo , severe air pollution problem P. M. Zhai13, N. Sugimoto15, S. S. Babu16, and G. P. Brasseur17 • Aerosol affects the Asian monsoon • Aerosol-monsoon interactions dictate 1State Key Laboratory of Earth Surface Processes and Resource Ecology and College of Global Change and Earth System the climate change in the region Science, Beijing Normal University, Beijing, China, 2Department of Atmospheric and Oceanic Science and ESSIC, University of Maryland,CollegePark,Maryland,USA, 3DepartmentofAtmosphericandClimateSciences,UniversityofCalifornia,SanDiego, California, USA, 4Institute of Atmospheric Physics, Chinese Academy of Sciences, Beijing, China, 5National Climate Center, Correspondence to: China Meteorological Administration, Beijing, China, 6Pacific Northwest National Laboratory, Richland, Washington, USA, Z. Li, 7Institute of Earth Sciences, Hebrew University, Jerusalem, Israel, 8Geophysical Fluid Dynamic Laboratory, NOAA, Princeton, [email protected] New Jersey, USA, 9Jet Propulsion Laboratory, California Institute of Technology, Pasadena, California, USA, 10College of Atmospheric Sciences, Lanzhou University, Lanzhou, China, 11Department of Atmospheric Sciences, University of Hawaii, Citation: Honolulu, Hawaii, USA, 12School of Atmospheric Physics, Nanjing University of Information Science and Technology, Nanjing, Li, Z., et al. -
Climate Impacts on Food Security and Livelihoods in Asia a Review of Existing Knowledge
Climate impacts on food security and livelihoods in Asia A review of existing knowledge WFP Bangladesh Chandan Roberto Ribeiro 2 Climate impacts on food security and livelihoods in Asia 1 Contents Introduction There is agreement in the scientific community that the global food system will experience unprecedented pressure in the coming decades – demographic changes, urban growth, environmental degradation, increasing disaster risk, food price volatility, and climate change will all affect food security patterns. 1 Introduction Climate change can act as a hunger Extreme weather effects disrupt the stability risk multiplier, exacerbating drivers of food of food supply as well as people’s livelihoods. 2 Asia is a diverse continent insecurity. Climate change disproportionately Understanding the specific impacts affects the poorest and most food insecure 4 Food security in Asia is changing of climate change on food security is through a combination of decreasing crop challenging because vulnerabilities are highly 6 Food security and livelihoods in Asia are sensitive to climate production, and changes in the frequency contextual and are unevenly spread across and intensity of climate-related hazards, the world. Ultimately, these vulnerabilities 11 The climate of Asia is driven by several processes all of which can result in more humanitarian also depend on the ability of households, and food security crises. communities, and countries to manage risks. 13 Climate is changing and will continue to change Climate change affects the different Under climate change, some regions of the 26 Climate and socioeconomic trends dimensions of food security in complex ways. world may experience gains in terms of The availability of food can be affected food security outcomes, but the poorest and 27 Key messages more isolated parts of the world tend to be through variations in yields – especially more adversely affected in the absence of in key producing areas – due to increasing 28 References adaptation efforts. -
The Origin of Asian Monsoons: a Modelling Perspective
1 The origin of Asian Monsoons: a modelling perspective 2 3 Delphine Tardif1, Frédéric Fluteau1, Yannick Donnadieu2, Guillaume Le Hir1, Jean-Baptiste Ladant3, 4 Pierre Sepulchre4, Alexis Licht5, Fernando Poblete6, Guillaume Dupont-Nivet7,8 5 6 1 Université de Paris, Institut de physique du globe de Paris, CNRS, 75005 Paris, France 7 2 Aix-Marseille Univ, CNRS, IRD, Coll France, INRA, CEREGE, Aix-en-Provence, France. 8 3 University of Michigan, Ann Arbor, MI, USA 9 4 Laboratoire des Sciences du Climat et de l’Environnement, LSCE/IPSL, CEA-CNRS-UVSQ, Université Paris-Saclay, 10 91191 Gif-sur-Yvette, France 11 5 University of Washington, Seattle, USA 12 6 Departamento de Geología, Universidad de Chile, Santiago, Chile 13 7 Univ. Rennes, CNRS, Géosciences Rennes, 35000 Rennes, France 14 8 Institute of Geosciences, Universität Potsdam, Germany 15 16 Abstract. The Cenozoic inception and development of the Asian monsoons remain unclear and have generated much 17 debate, as several hypotheses regarding circulation patterns at work in Asia during the Eocene have been proposed in the 18 last decades. These include a) the existence of modern-like monsoons since the early Eocene; b) that of a weak South 19 Asian Monsoon (SAM) and little to no East Asian Monsoon (EAM) or c) a prevalence of the Inter Tropical Convergence 20 Zone (ITCZ) migrations, also referred to as Indonesian-Australian Monsoon (I-AM). As SAM and EAM are supposed to 21 have been triggered or enhanced primarily by Asian paleogeographic changes, their possible inception in the very dynamic 22 Eocene paleogeographic context remains an open question, both in the modeling and field-based communities. -
Orientalism in Early Modern France Eurasian Trade, Exoticism, and the Ancien Régime
Orientalism in Early Modern France Eurasian Trade, Exoticism, and the Ancien Régime Ina Baghdiantz McCabe Oxford • New York Disclaimer: This eBook does not include the ancillary media that was packaged with the original printed version of the book. First published in 2008 by Berg Editorial offi ces: 1st Floor, Angel Court, 81 St Clements Street, Oxford, OX4 1AW, UK 175 Fifth Avenue, New York, NY 10010, USA © Ina Baghdiantz McCabe 2008 All rights reserved. No part of this publication may be reproduced in any form or by any means without the written permission of Berg. Berg is the imprint of Oxford International Publishers Ltd. Library of Congress Cataloguing-in-Publication Data McCabe, Ina Baghdiantz. Orientalism in early modern France : Eurasian trade, exoticism, and the Ancien Régime / Ina Baghdiantz McCabe. p. cm. Includes bibliographical references and index. ISBN-13: 978–1–84520–374–0 (cloth) ISBN-10: 1–84520–374–7 (cloth) 1. France—Civilization—Asian infl uences. 2. France—Relations— Asia. 3. Asia—Relations—France. 4. France—Foreign relations—1589– 1789. I. Title. DC33.3.M33 2008 303.48'2440509032—dc22 2008001197 British Library Cataloguing-in-Publication Data A catalogue record for this book is available from the British Library. ISBN 978 1 84520 374 0 (Cloth) Typeset by Apex Printed in the United Kingdom by Biddles Ltd, King’s Lynn www.bergpublishers.com Contents Acknowledgments v Introduction 1 PART I: ONE NATION, ONE WORLD UNDER FRENCH RULE 1 The First Orientalist, Guillaume Postel 15 2 The Ambassadors 37 3 France in the World