Band Filling and Cross Quantum Capacitance in Ion Gated Semiconducting Transition Metal Dichalcogenide Monolayers
Total Page:16
File Type:pdf, Size:1020Kb
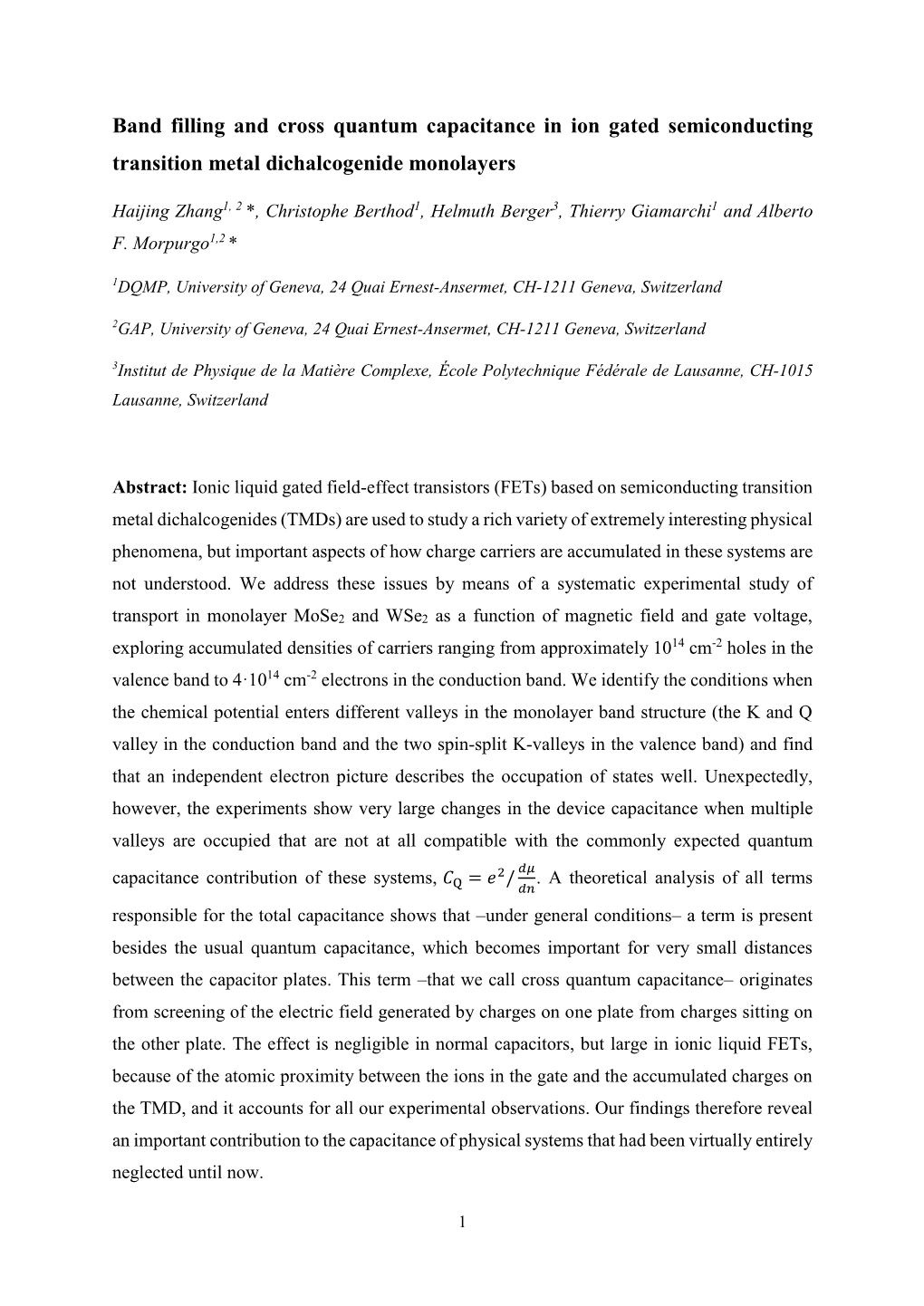
Load more
Recommended publications
-
An Intuitive Equivalent Circuit Model for Multilayer Van Der Waals Heterostructures Abhinandan Borah, Punnu Jose Sebastian, Ankur Nipane, and James T
1 An Intuitive Equivalent Circuit Model for Multilayer van der Waals Heterostructures Abhinandan Borah, Punnu Jose Sebastian, Ankur Nipane, and James T. Teherani Abstract—Stacks of 2D materials, known as van der Waals (vdW) heterostructures, have gained vast attention due to their Layer 1 interesting electrical and optoelectronic properties. This paper Dielectric 1 1 presents an intuitive circuit model for the out-of-plane electrostat- Layer 2 � ics of a vdW heterostructure composed of an arbitrary number of Dielectric 2 2D material / layers of 2D semiconductors, graphene, or metal. We explain the mapping between the out-of-plane energy-band diagram and the � metal elements of the equivalent circuit. Though the direct solution of n dielectric the circuit model for an -layer structure is not possible due to the Dielectric variable, nonlinear quantum capacitance of each layer, this work uses the intuition gained from the energy-band picture to provide Layer Dielectric � − an efficient solution in terms of a single variable. Our method �−1 employs Fermi-Dirac statistics while properly modeling the finite Layer � − � density of states (DOS) of 2D semiconductors and graphene. � − The approach circumvents difficulties that arise in commercial � �� TCAD tools, such as the proper handling of the 2D DOS and the simulation boundary conditions when the structure terminates Fig. 1. An n-layer vdW heterostructure with voltages applied to each layer. with a nonmetallic material. Based on this methodology, we have developed 2dmatstacks, an open-source tool freely available on nanohub.org. Overall, this work equips researchers to analyze, understand, and predict experimental results in complicated vdW [11], [13], [21]. -
Large Capacitance Enhancement in 2D Electron Systems Driven by Electron Correlations
Large capacitance enhancement in 2D electron systems driven by electron correlations Brian Skinner, University of Minnesota with B. I. Shklovskii May 4, 2013 Capacitance as a thermodynamic probe The differential capacitance material of interest V insulator contains information about thermodynamic properties metal electrode Correlated and quantum behavior can have dramatic manifestations in the capacitance. Thermodynamic definition of C Capacitance is determined by the total energy U(Q) -Q V +Q electrons Define the “effective capacitor thickness”: 2 2 d* = ε0ε/C = ε0ε (d U/dQ ) Thermodynamic definition of C 2D material with electron density n chemical potential μ -Q d d * d 0 V e2 dn +Q 1 1 1 C Cg Cq Quantum capacitance in 2D (Noninteracting) 2DEG with parabolic spectrum: dn const. m /2 d * d a / 4 d B graphene: 1/ 2 1/ 2 n Cq n n1/ 2 d * d 8 [Ponomarenko et. al., PRL 105, 136801 (2010)] -1 Electron correlations can make Cq large and negative, so that C >> Cg and d* << d In the remainder of this talk: 1. A 2D electron gas next to d a metal electrode V 2. Monolayer graphene in a strong magnetic field 2’. Double-layer graphene Large capacitance in a capacitor 1. with a conventional 2DEG A clean, gated 2DEG at zero temperature: electron density n(V) d 2DEG insulator V metal gate electrode What is d* = ε0ε/C as a function of n? Semiclassical scaling behavior -1/2 The problem has three length scales: d, aB << d, and n -1/2 case 1: n << aB 2DEG E d 2DEG is a degenerate Fermi gas ++++++++++++++++++++ metal d* = d + aB/4 Semiclassical scaling behavior -1/2 The problem has three length scales: d, aB << d, and n -1/2 -1/2 ~ n case 2: aB << n << d 2DEG Electrons undergo crystallization: E 2 1/2 2 ++++++++++++++++++++ e n /ε0ε >> h n/m metal electron repulsion >> kinetic energy Wigner crystal has negative density of states: d µ ~ -e2n1/2/ε ε d * d 0 0 e2 dn d* = d – 0.12 n-1/2 < d [Bello, Levin, Shklovskii, and Efros, Sov. -
Enhancement of Quantum Capacitance by Chemical Modification of Graphene Supercapacitor Electrodes: a Study by first Principles
Bull. Mater. Sci. (2019) 42:257 © Indian Academy of Sciences https://doi.org/10.1007/s12034-019-1952-8 Enhancement of quantum capacitance by chemical modification of graphene supercapacitor electrodes: a study by first principles TSRUTHI and KARTICK TARAFDER∗ Department of Physics, National Institute of Technology, Srinivasnagar, Surathkal, Mangalore 575025, India ∗Author for correspondence ([email protected]) MS received 23 October 2018; accepted 15 March 2019 Abstract. In this paper, we specify a powerful way to boost quantum capacitance of graphene-based electrode materials by density functional theory calculations. We performed functionalization of graphene to manifest high-quantum capacitance. A marked quantum capacitance of above 420 μFcm−2 has been observed. Our calculations show that quantum capacitance of graphene enhances with nitrogen concentration. We have also scrutinized effect on the increase of graphene quantum capacitance due to the variation of doping concentration, configuration change as well as co-doping with nitrogen and oxygen ad-atoms in pristine graphene sheets. A significant increase in quantum capacitance was theoretically detected in functionalized graphene, mainly because of the generation of new electronic states near the Dirac point and the shift of Fermi level caused by ad-atom adsorption. Keywords. Supercapacitor; quantum capacitance; density functional theory. 1. Introduction present study, we performed density functional theory (DFT) calculations to investigate quantum capacitance of function- Production of sufficient energy from renewable energy alized graphene by means of electronic structure calculations sources, bypassing the use of fossil fuels, natural gas and coal [5]. We explored the impact of increasing nitrogen and oxy- is one of the biggest challenges to suppress climate change. -
The Role of Band Structure and Quantum Capacitance Iddo Heller, Jing Kong,† Keith A
Published on Web 05/12/2006 Electrochemistry at Single-Walled Carbon Nanotubes: The Role of Band Structure and Quantum Capacitance Iddo Heller, Jing Kong,† Keith A. Williams,‡ Cees Dekker, and Serge G. Lemay* Contribution from the KaVli Institute of Nanoscience, Delft UniVersity of Technology, Lorentzweg 1, 2628 CJ Delft, The Netherlands Received February 20, 2006; E-mail: [email protected] Abstract: We present a theoretical description of the kinetics of electrochemical charge transfer at single- walled carbon nanotube (SWNT) electrodes, explicitly taking into account the SWNT electronic band structure. SWNTs have a distinct and low density of electronic states (DOS), as expressed by a small value of the quantum capacitance. We show that this greatly affects the alignment and occupation of electronic states in voltammetric experiments and thus the electrode kinetics. We model electrochemistry at metallic and semiconducting SWNTs as well as at graphene by applying the Gerischer-Marcus model of electron transfer kinetics. We predict that the semiconducting or metallic SWNT band structure and its distinct van Hove singularities can be resolved in voltammetry, in a manner analogous to scanning tunneling spectroscopy. Consequently, SWNTs of different atomic structure yield different rate constants due to structure-dependent variations in the DOS. Interestingly, the rate of charge transfer does not necessarily vanish in the band gap of a semiconducting SWNT, due to significant contributions from states which are a few kBT away from the Fermi level. The combination of a nanometer critical dimension and the distinct band structure makes SWNTs a model system for studying the effect of the electronic structure of the electrode on electrochemical charge transfer. -
Mobility Extraction and Quantum Capacitance Impact in High Performance Graphene Field-Effect Transistor Devices
Mobility Extraction and Quantum Capacitance Impact in High Performance Graphene Field-effect Transistor Devices Zhihong Chen1, Joerg Appenzeller2 1 IBM T.J. Watson Research Center, Yorktown Heights, NY 10598, USA, 2 School of Electrical and Computer Engineering and Birck Nanotechnology Center, Purdue University, West Lafayette, IN 47907, USA Abstract transconductance per channel width (gm/W) (top of Fig. 2). The field-effect mobility of graphene devices is discussed. For L>2μm on the other hand, gm/W decreases We argue that the graphene ballistic mean free path, Lball can only be extracted by taking into account both, the electrical characteristics and the channel length dependent mobility. In doing so we find a ballistic mean free path of Lball=300±100nm at room-temperature for a carrier concentration of ~1012cm-2 and that a substantial series resistance of around 300Ωμm has to be taken into account. Furthermore, we demonstrate first quantum capacitance measurements on single-layer graphene devices. Introduction With the need to ever further improve the performance of field-effect transistors (FETs) researchers are evaluating various novel channel materials. The most recent candidate that has sparked a substantial amount of excitement is graphene. Graphene occurs to be an ideal choice for FET Fig. 1: Scanning electron microscopy images of applications since it combines a) an ultra-thin body for back-gated graphene field-effect transistors with aggressive channel length scaling with b) excellent various channel lengths and widths. intrinsic transport properties [1-4] similar to carbon nanotubes but with c) the chance to pattern the desired monotonically with increasing L as evident from the device structures within a top-down lithographical bottom part of Fig. -
Direct Measurement of Discrete Valley and Orbital Quantum Numbers in a Multicomponent Quantum Hall System
Direct measurement of discrete valley and orbital quantum numbers in a multicomponent quantum Hall system B.M. Hunt1;2;3, J.I.A. Li2, A.A. Zibrov4, L. Wang5, T. Taniguchi6, K. Watanabe6, J. Hone5, C. R. Dean2, M. Zaletel7, R.C. Ashoori1, A.F. Young1;4;∗ 1Department of Physics, Massachusetts Institute of Technology, Cambridge, MA 02139 2Department of Physics, Columbia University, New York, NY 10027, USA 3Department of Physics, Carnegie Mellon University, Pittsburgh, Pennsylvania 15213, USA 5Department of Mechanical Engineering, Columbia University, New York, NY 10027, USA 6Advanced Materials Laboratory, National Institute for Materials Science, Tsukuba, Ibaraki 305-0044, Japan 7Station Q, Microsoft Research, Santa Barbara, California 93106-6105, USA 4Department of Physics, University of California, Santa Barbara CA 93106 USA ∗[email protected] Strongly interacting two dimensional electron systems ence of internal degeneracy. The Bernal bilayer graphene (B- (2DESs) host a complex landscape of broken symmetry BLG) zero energy Landau level (ZLL) provides an extreme states. The possible ground states are further expanded by example of such degeneracy. In B-BLG, LLs jξNσi are la- internal degrees of freedom such as spin or valley-isospin. beled by their electron spin σ ="; #, valley ξ = +; −, and While direct probes of spin in 2DESs were demonstrated “orbital” index N 2 Z. Electrons in valley +=− are local- two decades ago1, the valley quantum number has only ized near points K=K0 of the hexagonal Brillouin zone, while been probed indirectly in semiconductor quantum wells2, the index N is closely analogous to the LL-index of conven- graphene mono-3,4 and bilayers5–13, and, transition metal tional LL systems. -
UNIVERSITY of CALIFORNIA RIVERSIDE Modeling, Design, And
UNIVERSITY OF CALIFORNIA RIVERSIDE Modeling, Design, and Analysis of III-V Nanowire Transistors and Tunneling Transistors A Dissertation submitted in partial satisfaction of the requirements for the degree of Doctor of Philosophy in Electrical Engineering by Mohammad Abul Khayer March 2011 Dissertation Committee: Dr. Roger K. Lake, Chairperson Dr. Alexander A. Balandin Dr. Javier E. Garay Copyright by Mohammad Abul Khayer 2011 The Dissertation of Mohammad Abul Khayer is approved: Committee Chairperson University of California, Riverside Acknowledgments At first, I would like to express my heartiest gratitude and deep appreciation to my PhD advisor Professor Roger Lake, Department of Electrical Engineering, University of California at Riverside, for his continuous support, interactive guidance and sug- gestions during the past four years as his student. Without his strenuous engagement in this thesis, this work would not be possible to materialize. My experience of work- ing and interacting with Prof. Lake on developing modeling and simulation tools, analytical models, deriving theory, understanding and solving the crucial problems for nanoscale devices is invaluable. I am grateful to my Doctoral committee members for their support and suggestions. I appreciate discussions with my former lab mates Dr. Khairul Alam and Dr. Nicolas Bruque. To my family: I thank to my mom (Amma), brothers (Elias, Delwar, Shahid), sisters (Saleha and Rehana) for their support throughout graduate school. And lastly, to my dearest and loving wife Sohana, who has dealt with all the ups and downs by supporting and encouraging me without any condition, while pursuing her PhD in Mechanical Engineering. I would be delighted to convey my special thanks to my cute and beautiful son Aayan, whom while taking in my lap I was being able to work in my laptop. -
Gate Electrostatics and Quantum Capacitance in Ballistic Graphene
Gate electrostatics and quantum capacitance in ballistic graphene devices José M. Caridad 1† , Stephen R. Power 2,3,4 , Artsem A. Shylau 1, Lene Gammelgaard 1, Antti-Pekka Jauho 1, Peter Bøggild 1 1Center for Nanostructured Graphene (CNG), Department of Physics, Technical University of Denmark, 2800 Kongens Lyngby, Denmark 2Catalan Institute of Nanoscience and Nanotechnology (ICN2), CSIC and The Barcelona Institute of Science and Technology, Campus UAB, Bellaterra, 08193 Barcelona, Spain 3Universitat Autònoma de Barcelona, 08193 Bellaterra (Cerdanyola del Vallès), Spain 4School of Physics, Trinity College Dublin, Dublin 2, Ireland †corresponding author: [email protected] ABSTRACT. We experimentally investigate the charge induction mechanism across gated, narrow, ballistic graphene devices with different degrees of edge disorder. By using magnetoconductance measurements as the probing technique, we demonstrate that devices with large edge disorder exhibit a nearly homogeneous capacitance profile across the device channel, close to the case of an infinitely large graphene sheet. In contrast, devices with lower edge disorder (< 1 nm roughness) are strongly influenced by the fringing electrostatic field at graphene boundaries, in quantitative agreement with theoretical calculations for pristine systems. Specifically, devices with low edge disorder present a large effective capacitance variation across the device channel with a nontrivial, inhomogeneous profile due not only to 1 classical electrostatics but also to quantum mechanical effects. We show that such quantum capacitance contribution, occurring due to the low density of states (DOS) across the device in the presence of an external magnetic field, is considerably altered as a result of the gate electrostatics in the ballistic graphene device. Our conclusions can be extended to any two- dimensional (2D) electronic system confined by a hard-wall potential and are important for understanding the electronic structure and device applications of conducting 2D materials. -
UNIVERSITY of CALIFORNIA RIVERSIDE Computational Insights
UNIVERSITY OF CALIFORNIA RIVERSIDE Computational Insights into Materials and Interfaces for Capacitive Energy Storage A Dissertation submitted in partial satisfaction of the requirements for the degree of Doctor of Philosophy in Chemistry by Cheng Zhan June 2018 Dissertation Committee: Dr. De-en Jiang, Chairperson Dr. Gregory Beran Dr. Ludwig Bartels Copyright by Cheng Zhan June 2018 The Dissertation of Cheng Zhan is approved: Committee Chairperson University of California, Riverside COPYRIGHT ACKNOWLEDGEMENT The text and figures in Chapter 1, Chapter 2 and Chapter 10, in part or full, are a reprint of the material as it appears in Adv. Sci., 4, 170059 (2017). The coauthor (Dr. De-en Jiang) listed in that publication directed and supervised the research that forms the basis of this chapter. The text and figures in Chapter 3, in part or full, are a reprint of the material as it appears in J. Phys. Chem. C, 119, 22297–22303 (2015). The coauthor (Dr. De-en Jiang) listed in that publication directed and supervised the research that forms the basis of this chapter. The text and figures in Chapter 4, in part or full, are a reprint of the material as it appears in Phys. Chem. Chem. Phys., 18, 4668-4674 (2016). The coauthor (Dr. De-en Jiang) listed in that publication directed and supervised the research that forms the basis of this chapter. The text and figures in Chapter 5, in part or full, are a reprint of the material as it appears in J. Phys. Chem. Lett., 7, 789−794 (2016). The coauthor (Dr. De-en Jiang) listed in that publication directed and supervised the research that forms the basis of this chapter. -
Gate Quantum Capacitance Effects in Nanoscale Transistors
Letter Cite This: Nano Lett. 2019, 19, 7130−7137 pubs.acs.org/NanoLett Gate Quantum Capacitance Effects in Nanoscale Transistors † ‡ § † ‡ § † ∥ † ‡ § Sujay B. Desai, , , Hossain M. Fahad, , , Theodor Lundberg, Gregory Pitner, Hyungjin Kim, , , ‡ ⊥ ∥ † ‡ § Daryl Chrzan, , H.-S. Philip Wong, and Ali Javey*, , , † Electrical Engineering and Computer Sciences, University of California, Berkeley, Berkeley, California 94720, United States ‡ Materials Sciences Division, Lawrence Berkeley National Laboratory, Berkeley, California 94720, United States § Berkeley Sensor and Actuator Center, University of California, Berkeley, Berkeley, California 94720, United States ∥ Electrical Engineering, Stanford University, Stanford, California 94305, United States ⊥ Materials Science and Engineering Department, University of California, Berkeley, Berkeley, California 94720, United States *S Supporting Information ABSTRACT: As the physical dimensions of a transistor gate continue to shrink to a few atoms, performance can be increasingly determined by the limited electronic density of states (DOS) in the gate and the gate quantum capacitance (CQ). We demonstrate the impact of gate CQ and the dimensionality of the gate electrode on the performance of nanoscale transistors through analytical electrostatics model- ing. For low-dimensional gates, the gate charge can limit the channel charge, and the transfer characteristics of the device become dependent on the gate DOS. We experimentally observe for the first time, room-temperature gate quantization features in the transfer characteristics of single-walled carbon nanotube (CNT)-gated ultrathin silicon-on-insulator (SOI) channel transistors; features which can be attributed to the Van Hove singularities in the one-dimensional DOS of the CNT gate. In addition to being an important aspect of future transistor design, potential applications of this phenomenon include multilevel transistors with suitable transfer characteristics obtained via engineered gate DOS. -
Compressibility and Capacitance of Quantum Systems
Compressibility and Capacitance of Quantum Systems Dissertation zur Erlangung des akademischen Grades Dr. rer. nat. eingereicht an der Mathematisch-Naturwissenschaftlich-Technischen Fakult¨at der Universit¨atAugsburg von Kevin Patrick Steffen Augsburg, Juli 2017 Tag der m¨undlichen Pr¨ufung: 04. September 2017 Erstgutachter: Prof. Dr. Thilo Kopp Zweitgutachter: Prof. Dr. Gert-Ludwig Ingold Contents 1. Prologue 1 2. Introduction to Capacitance and Compressibility 5 2.1. Compressibility of a Thermodynamic System . .5 2.2. Coupled Electron Systems . .8 2.3. Compressibility and its Dependence on the Distribution of the Positive Back- ground Charge . 15 2.4. Experimental Findings of Negative Capacitance and Compressibility of Subsystems 20 2.5. Further Theoretical Approaches to Capacitance Analysis . 23 3. Exchange and Correlation Contributions at Finite Temperature 25 3.1. Hartree-Fock Approximation . 25 3.2. Groundstate of the Free Electron Gas . 31 3.3. Correlation Energy and Second Derivatives with Respect to Density . 32 3.4. Uniform Electron Gas and its Compressibility at Finite Temperature . 35 4. Density Dependent Spin-Orbit Coupling 43 4.1. Spin-Orbit Coupling . 43 4.2. Density Dependent Rashba Spin-Orbit Coupling . 44 4.2.1. Comparison of Parameters to Experiment . 47 4.2.2. Compressibility and Capacitance . 48 4.2.3. Capacitance of a System with Three-Dimensional Top Electrode . 53 4.3. Six-Band Model of a Layer with Spin-Orbit Coupling . 55 4.3.1. Introduction to the Model . 55 4.3.2. Capacitance and Compressibility for Different Electrostatic Layouts . 59 4.4. Summary of the Section . 65 5. Electron System with Rashba Spin-Orbit Coupling and Exchange Interaction 67 5.1. -
Quantum Capacitance of Coupled Two-Dimensional Electron Gases
Journal of Physics: Condensed Matter ACCEPTED MANUSCRIPT Quantum Capacitance of Coupled Two-Dimensional Electron Gases To cite this article before publication: krishna bharadwaj Balasubramanian 2021 J. Phys.: Condens. Matter in press https://doi.org/10.1088/1361- 648X/abe64f Manuscript version: Accepted Manuscript Accepted Manuscript is “the version of the article accepted for publication including all changes made as a result of the peer review process, and which may also include the addition to the article by IOP Publishing of a header, an article ID, a cover sheet and/or an ‘Accepted Manuscript’ watermark, but excluding any other editing, typesetting or other changes made by IOP Publishing and/or its licensors” This Accepted Manuscript is © 2021 IOP Publishing Ltd. During the embargo period (the 12 month period from the publication of the Version of Record of this article), the Accepted Manuscript is fully protected by copyright and cannot be reused or reposted elsewhere. As the Version of Record of this article is going to be / has been published on a subscription basis, this Accepted Manuscript is available for reuse under a CC BY-NC-ND 3.0 licence after the 12 month embargo period. After the embargo period, everyone is permitted to use copy and redistribute this article for non-commercial purposes only, provided that they adhere to all the terms of the licence https://creativecommons.org/licences/by-nc-nd/3.0 Although reasonable endeavours have been taken to obtain all necessary permissions from third parties to include their copyrighted content within this article, their full citation and copyright line may not be present in this Accepted Manuscript version.