Maintaining Trunk Neural Crest Cells As Crestospheres
Total Page:16
File Type:pdf, Size:1020Kb
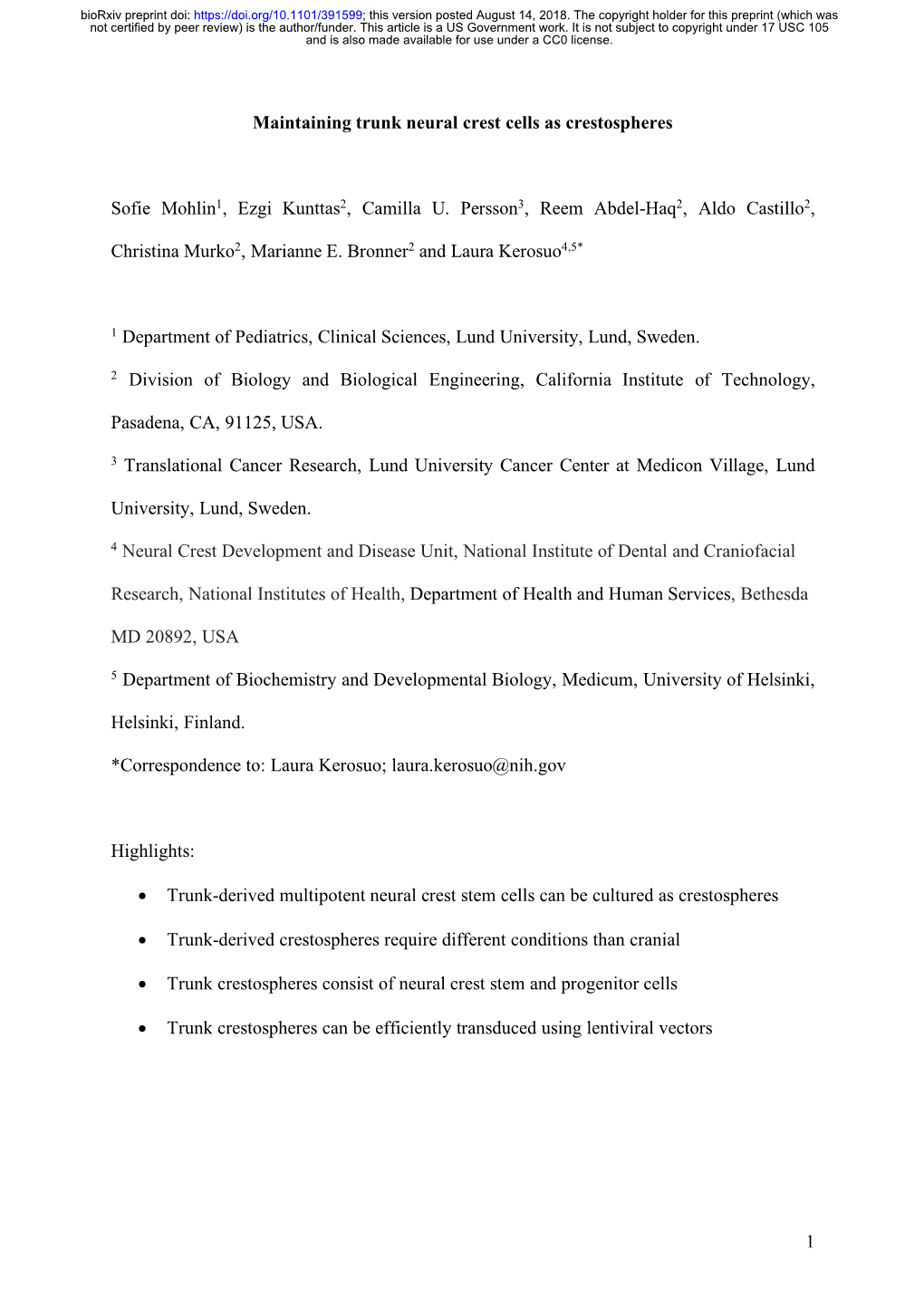
Load more
Recommended publications
-
And Late-Migrating Cranial Neural Crest Cell Populations Have Equivalent Developmental Potential in Vivo
Development 124, 3077-3087 (1997) 3077 Printed in Great Britain © The Company of Biologists Limited 1997 DEV3724 Early- and late-migrating cranial neural crest cell populations have equivalent developmental potential in vivo Clare V. H. Baker1,2,*, Marianne Bronner-Fraser1, Nicole M. Le Douarin2 and Marie-Aimée Teillet2 1Division of Biology, Beckman Institute 139-74, California Institute of Technology, Pasadena, California 91125, USA 2Institut d’Embryologie cellulaire et moléculaire du CNRS et du Collège de France, 49bis avenue de la Belle Gabrielle, 94736 Nogent-sur-Marne Cedex, France *Author for correspondence currently at address1 SUMMARY We present the first in vivo study of the long-term fate and heterochronically for the late-migrating population, it no potential of early-migrating and late-migrating mesen- longer contributes to the jaw skeleton and only forms cephalic neural crest cell populations, by performing dorsal derivatives. When the late-migrating population is isochronic and heterochronic quail-to-chick grafts. Both grafted into a late-stage host whose neural crest had previ- early- and late-migrating populations form melanocytes, ously been ablated, it migrates ventrally into the jaws. neurons, glia, cartilage and bone in isochronic, isotopic Thus, the dorsal fate restriction of the late-migrating mes- chimeras, showing that neither population is lineage- encephalic neural crest cell population in normal develop- restricted. The early-migrating population distributes both ment is due to the presence of earlier-migrating neural dorsally and ventrally during normal development, while crest cells, rather than to any change in the environment or the late-migrating population is confined dorsally and to any intrinsic difference in migratory ability or potential forms much less cartilage and bone. -
Migratory Patterns and Developmental Potential of Trunk Neural Crest Cells in the Axolotl Embryo
DEVELOPMENTAL DYNAMICS 236:389–403, 2007 RESEARCH ARTICLE Migratory Patterns and Developmental Potential of Trunk Neural Crest Cells in the Axolotl Embryo Hans-Henning Epperlein,1* Mark A.J. Selleck,2 Daniel Meulemans,3 Levan Mchedlishvili,4 Robert Cerny,5 Lidia Sobkow,4 and Marianne Bronner-Fraser3 Using cell markers and grafting, we examined the timing of migration and developmental potential of trunk neural crest cells in axolotl. No obvious differences in pathway choice were noted for DiI-labeling at different lateral or medial positions of the trunk neural folds in neurulae, which contributed not only to neural crest but also to Rohon-Beard neurons. Labeling wild-type dorsal trunks at pre- and early-migratory stages revealed that individual neural crest cells migrate away from the neural tube along two main routes: first, dorsolaterally between the epidermis and somites and, later, ventromedially between the somites and neural tube/notochord. Dorsolaterally migrating crest primarily forms pigment cells, with those from anterior (but not mid or posterior) trunk neural folds also contributing glia and neurons to the lateral line. White mutants have impaired dorsolateral but normal ventromedial migration. At late migratory stages, most labeled cells move along the ventromedial pathway or into the dorsal fin. Contrasting with other anamniotes, axolotl has a minor neural crest contribution to the dorsal fin, most of which arises from the dermomyotome. Taken together, the results reveal stereotypic migration and differentiation of neural crest cells in axolotl that differ from other vertebrates in timing of entry onto the dorsolateral pathway and extent of contribution to some derivatives. -
Migration and Proliferation of Cultured Neural Crest Cells in W Mutant Neural Crest Chimeras
Development 112, 131-141 (1991) 131 Printed in Great Britain © The Company of Biologists Limited 1991 Migration and proliferation of cultured neural crest cells in W mutant neural crest chimeras D. HUSZAR*, A. SHARPE and R. JAENISCH Whitehead Institute for Biomedical Research, Nine Cambridge Center, Cambridge, MA 02142, USA and Department of Biology, Massachusetts Institute of Technology, Cambridge, MA 02139, USA •Present address GenPharm International, 2375 Garcia Avenue, Mountain View, CA 94043, USA Summary Chimeric mice, generated by aggregating preimplan- functional endogenous melanoblast population in W tation embryos, have been instrumental in the study of mutants, in contrast to Balb/c mice, which contain a full the development of coat color patterns in mammals. This complement of melanocytes. Our results suggest that the approach, however, does not allow for direct experimen- W mutation disturbs migration and/or proliferation of tal manipulation of the neural crest cells, which are the endogenous melanoblasts. In order to obtain infor- precursors of melanoblasts. We have devised a system mation on clonal size and extent of intermingling of that allows assessment of the developmental potential donor cells, two genetically marked neural crest cell and migration of neural crest cells in vivo following their populations were mixed and coinjected into W embryos. experimental manipulation in vitro. Cultured C57B1/6 In hau* of the tricolored chimeras, no co-localization of neural crest cells were microinjected in utero into donor crest cells was observed, while, in the other half, a neurulating Balb/c or W embryos and shown to fine intermingling of donor-derived colors had occurred. contribute efficiently to pigmentation in the host animal. -
Specification and Formation of the Neural Crest: Perspectives on Lineage Segregation
Received: 3 November 2018 Revised: 17 December 2018 Accepted: 18 December 2018 DOI: 10.1002/dvg.23276 REVIEW Specification and formation of the neural crest: Perspectives on lineage segregation Maneeshi S. Prasad1 | Rebekah M. Charney1 | Martín I. García-Castro Division of Biomedical Sciences, School of Medicine, University of California, Riverside, Summary California The neural crest is a fascinating embryonic population unique to vertebrates that is endowed Correspondence with remarkable differentiation capacity. Thought to originate from ectodermal tissue, neural Martín I. García-Castro, Division of Biomedical crest cells generate neurons and glia of the peripheral nervous system, and melanocytes Sciences, School of Medicine, University of California, Riverside, CA. throughout the body. However, the neural crest also generates many ectomesenchymal deriva- Email: [email protected] tives in the cranial region, including cell types considered to be of mesodermal origin such as Funding information cartilage, bone, and adipose tissue. These ectomesenchymal derivatives play a critical role in the National Institute of Dental and Craniofacial formation of the vertebrate head, and are thought to be a key attribute at the center of verte- Research, Grant/Award Numbers: brate evolution and diversity. Further, aberrant neural crest cell development and differentiation R01DE017914, F32DE027862 is the root cause of many human pathologies, including cancers, rare syndromes, and birth mal- formations. In this review, we discuss the current -
Migration and Differentiation of Neural Crest and Ventral Neural Tube Cells in Vitro: Implications for in Vitro and in Vivo Studies of the Neural Crest
The Journal of Neuroscience, March 1988, 8(3): 1001-l 01.5 Migration and Differentiation of Neural Crest and Ventral Neural Tube Cells in vitro: Implications for in vitro and in vivo Studies of the Neural Crest J. F. Loring,’ D. L. Barker;,= and C. A. Erickson’ ‘Department of Zoology, University of California, Davis, California 95616, and *Hatfield Marine Sciences Center, Newport, Oregon 97365 During vertebrate development, neural crest cells migrate trunk neural crest cell cultures, a number of classicalneuro- from the dorsal neural tube and give rise to pigment cells transmitters have been reported, including catecholamines(Co- and most peripheral ganglia. To study these complex pro- hen, 1977; Loring et al., 1982;Maxwell et al., 1982) ACh (Kahn cesses it is helpful to make use of in vitro techniques, but et al., 1980; Maxwell et al., 1982) GABA (Maxwell et al., 1982), the transient and morphologically ill-defined nature of neural and 5-HT (Sieber-Blum et al., 1983). In addition, neuroactive crest cells makes it difficult to isolate a pure population of peptides, suchas somatostatin (Maxwell et al., 1984) have been undifferentiated cells. We have used several established reported in neural crest cell culture. techniques to obtain neural crest-containing cultures from In spite of the advantagesof an in vitro approachto analysis quail embryos and have compared their subsequent differ- of neural crest differentiation, it is clear that a fundamental entiation. We confirm earlier reports of neural crest cell dif- problem ariseswhen attempts are made to isolate these cellsin ferentiation in vitro into pigment cells and catecholamine- culture. -
Significance of Neural Crest in Tooth Development
OMPJ VP Jayasekharan et al 10.5005/jp-journals-10037-1018 REVIEW ARTICLE Signifi cance of Neural Crest in Tooth Development: The Molecular Signature 1VP Jayasekharan, 2Jacob Kurien, 3Eapen Cherian, 4Renji K Paul, 5Aravind S Raju ABSTRACT cells to those of mesenchymal cells. In the head region, The neural crest originates from cells located along the lateral incipient neural crest cells send out processes that penetrate margins of the neural plate. Neural crest cells arise as the result the basal lamina underlying the neuroepithelium just before of an inductive action by the non-neural ectoderm adjacent to neural tube closure. the neural plate and possibly by nearby mesoderm as well. As the neural tube forms, a group of cells separate from the neuro- The neural crest provides cells for the development ectoderm. These cells have the capacity to migrate and differen- of future organs and tissues. After the induction of neural tiate extensively within the developing embryo and they are the crest, the neural crest cells are formed within neural plate basis of structures such as spinal sensory ganglia, sympathetic border regions, which rise as neural folds, and ultimately neurons, Schwann cells, pigment cells and meninges. Specifi c interactions occur during the development of tooth and recent converge to form the dorsal midline of the neural tube, and research has concentrated more on the molecular aspects of it is from here that the neural crest cells will emerge in an these interactions. Thus, it is highly imperative to understand and anterior to posterior sequence. During and after migration, digress the complex mechanisms involved in these processes. -
Developmental Potential of Trunk Neural Crest Cells in the Mouse
Development 120, 1709-1718 (1994) 1709 Printed in Great Britain © The Company of Biologists Limited 1994 Developmental potential of trunk neural crest cells in the mouse George N. Serbedzija1,†, Marianne Bronner-Fraser2 and Scott E. Fraser1,* 1Division of Biology, California Institute of Technology, Pasadena, CA 91125, USA 2Developmental Biology Center, University of California at Irvine, Irvine, CA 92717, USA *Author for correspondence †Present address: Cellular and Developmental Biology, Harvard University, 16 Divinity Avenue, Cambridge, MA 02138, USA SUMMARY The availability of naturally occurring and engineered derivatives. The time of injection influenced the derivatives mutations in mice which affect the neural crest makes the populated by the labeled cells. Injections at early stages of mouse embryo an important experimental system for migration yielded labeled progeny in both dorsal and studying neural crest cell differentiation. Here, we ventral neural crest derivatives, whereas those performed determine the normal developmental potential of neural at later stages had labeled cells only in more dorsal neural crest cells by performing in situ cell lineage analysis in the crest derivatives, such as dorsal root ganglion and pre- mouse by microinjecting lysinated rhodamine dextran sumptive pigment cells. The results suggest that in the (LRD) into individual dorsal neural tube cells in the trunk. mouse embryo: (1) there is a common precursor for neural Labeled progeny derived from single cells were found in crest and neural tube cells; (2) some neural crest cells are the neural tube, dorsal root ganglia, sympathoadrenal multipotent; and (3) the timing of emigration influences the derivatives, presumptive Schwann cells and/or pigment range of possible neural crest derivatives. -
Ectoderm: Neurulation, Neural Tube, Neural Crest
4. ECTODERM: NEURULATION, NEURAL TUBE, NEURAL CREST Dr. Taube P. Rothman P&S 12-520 [email protected] 212-305-7930 Recommended Reading: Larsen Human Embryology, 3rd Edition, pp. 85-102, 126-130 Summary: In this lecture, we will first consider the induction of the neural plate and the formation of the neural tube, the rudiment of the central nervous system (CNS). The anterior portion of the neural tube gives rise to the brain, the more caudal portion gives rise to the spinal cord. We will see how the requisite numbers of neural progenitors are generated in the CNS and when these cells become post mitotic. The molecular signals required for their survival and further development will also be discussed. We will then turn our attention to the neural crest, a transient structure that develops at the site where the neural tube and future epidermis meet. After delaminating from the neuraxis, the crest cells migrate via specific pathways to distant targets in an embryo where they express appropriate target-related phenotypes. The progressive restriction of the developmental potential of crest-derived cells will then be considered. Additional topics include formation of the fundamental subdivisions of the CNS and PNS, as well as molecular factors that regulate neural induction and regional distinctions in the nervous system. Learning Objectives: At the conclusion of the lecture you should be able to: 1. Discuss the tissue, cellular, and molecular basis for neural induction and neural tube formation. Be able to provide some examples of neural tube defects caused by perturbation of neural tube closure. -
Neural Crest [1]
Published on The Embryo Project Encyclopedia (https://embryo.asu.edu) Neural Crest [1] By: Barnes, M. Elizabeth Keywords: Arthur Marshall [2] Julia Platt [3] neural crest cells [4] neurocristopathies [5] Early in the process of development, vertebrate embryos develop a fold on then eural plate [6] where the neural and epidermal ectoderms meet, called the neural crest [7]. The neural crest [7] produces neural crest cells [8] (NCCs), which become multiple different cell types and contribute to tissues and organs as an embryo develops. A few of the organs and tissues include peripheral and enteric (gastrointestinal) neurons and glia [9], pigment cells, cartilage and bone of the cranium and face, and smooth muscle. The diversity of NCCs that the neural crest [7] produces has led researchers to propose the neural crest [7] as a fourth germ layer, or one of the primary cellular structures in early embryos from which all adult tissues and organs arise. Furthermore, evolutionary biologists study the neural crest [7] because it is a novel shared evolutionary character (synapomorphy) of all vertebrates. Although the neural crest [7] first appears in the embryo during gastrulation [10], the invagination and spreading process by which a blastula [11] becomes a gastrula [12], it becomes distinguishable during the neurula stage. The neurula-stage of development occurs when the neural plate folds and transforms into the neural tube [13], the structure that will eventually develop into the central nervous system [14]. The neural crest arises at two junctions, one on each side of the midline of then eural plate [6], between neural and non-neural ectoderm [15]. -
Guidance of Trunk Neural Crest Migration Requires Neuropilin 2
RESEARCH ARTICLE 99 Development 133, 99-106 doi:10.1242/dev.02187 Guidance of trunk neural crest migration requires neuropilin 2/semaphorin 3F signaling Laura S. Gammill1, Constanza Gonzalez1, Chenghua Gu2,3 and Marianne Bronner-Fraser1,* In vertebrate embryos, neural crest cells migrate only through the anterior half of each somite while avoiding the posterior half. We demonstrate that neural crest cells express the receptor neuropilin 2 (Npn2), while its repulsive ligand semaphorin 3F (Sema3f) is restricted to the posterior-half somite. In Npn2 and Sema3f mutant mice, neural crest cells lose their segmental migration pattern and instead migrate as a uniform sheet, although somite polarity itself remains unchanged. Furthermore, Npn2 is cell autonomously required for neural crest cells to avoid Sema3f in vitro. These data show that Npn2/Sema3f signaling guides neural crest migration through the somite. Interestingly, neural crest cells still condense into segmentally arranged dorsal root ganglia in Npn2 nulls, suggesting that segmental neural crest migration and segmentation of the peripheral nervous system are separable processes. KEY WORDS: Trunk neural crest migration, Sclerotome, Neuropilin 2, Semaphorin 3F, Mouse, Chick INTRODUCTION Likewise, neuropilin 1 and its ligand semaphorin 3A have been The neural crest is a multipotent population of migratory cells that suggested to play a role (Eickholt et al., 1999), but are not expressed gives rise to a wide variety of different lineages in vertebrates. at the right time (reviewed by Kuan et al., 2004) and are not required During development, neural crest cells arise in the central nervous in the mouse for appropriate trunk neural crest migration (Kawasaki system, but subsequently migrate away and follow defined et al., 2002). -
The Neural Crest and Craniofacial Malformations
Chapter 5 The Neural Crest and Craniofacial Malformations Hans J.ten Donkelaar and Christl Vermeij-Keers 5.1 Introduction the head (Vermeij-Keers 1990; Sulik 1996; LaBonne and Bronner-Fraser 1999; Le Douarin and Kalcheim The neural crest is a temporary embryonic structure 1999; Sperber 2002; Knecht and Bronner-Fraser 2002; that is composed of a population of multipotent cells Francis-West et al. 2003; Santagati and Rijli 2003). In that delaminate from the ectoderm by epitheliomes- addition, during later developmental stages multiple enchymal tranformation (EMT; Duband et al. 1995; places of EMT are recognized as well. In the head– Hay 1995; Le Douarin and Kalcheim 1999; Francis- neck area, for example, the neurogenic placodes and West et al. 2003). Neural-crest-derived cells are called the optic neural crest are such areas. Neurogenic pla- mesectodermal or ectomesenchymal cells (mesoder- codes, specialized regions of the embryonic ecto- mal cells of ectodermal origin) that have arisen derm, are the major source of primary sensory neu- through EMT. The neural crest was first described rons in the head (Johnston and Bronsky 1995; Gra- by His (1868) in the chick embryo as a Zwischen- ham and Begbie 2000). The vasculature of the head is strang, a strip of cells lying between the dorsal ecto- derived from mesoderm-derived endothelial precur- derm and the neural tube. Classic contributions in sors,while the neural crest provides the pericytes and amphibians identified interactions between tissues smooth muscle cells of the vessels of the face and the that lead to neural crest formation, and were re- forebrain (Etchevers et al. -
The Cardiac Neural Crest Cells in Heart Development and Congenital Heart Defects
Journal of Cardiovascular Development and Disease Review The Cardiac Neural Crest Cells in Heart Development and Congenital Heart Defects Shannon Erhardt 1,2, Mingjie Zheng 1 , Xiaolei Zhao 1 , Tram P. Le 1 , Tina O. Findley 1 and Jun Wang 1,2,* 1 Department of Pediatrics, McGovern Medical School at UTHealth, The University of Texas Health Science Center at Houston, Houston, TX 77030, USA; [email protected] (S.E.); [email protected] (M.Z.); [email protected] (X.Z.); [email protected] (T.P.L.); tina.o.fi[email protected] (T.O.F.) 2 The University of Texas MD Anderson Cancer Center UTHealth Graduate School of Biomedical Sciences, The University of Texas Health Science Center at Houston, Houston, TX 77030, USA * Correspondence: [email protected] Abstract: The neural crest (NC) is a multipotent and temporarily migratory cell population stemming from the dorsal neural tube during vertebrate embryogenesis. Cardiac neural crest cells (NCCs), a specified subpopulation of the NC, are vital for normal cardiovascular development, as they signifi- cantly contribute to the pharyngeal arch arteries, the developing cardiac outflow tract (OFT), cardiac valves, and interventricular septum. Various signaling pathways are shown to orchestrate the proper migration, compaction, and differentiation of cardiac NCCs during cardiovascular development. Any loss or dysregulation of signaling pathways in cardiac NCCs can lead to abnormal cardiovascular development during embryogenesis, resulting in abnormalities categorized as congenital heart de- fects (CHDs). This review focuses on the contributions of cardiac NCCs to cardiovascular formation, discusses cardiac defects caused by a disruption of various regulatory factors, and summarizes the role of multiple signaling pathways during embryonic development.