Fuel Cell Technology
Total Page:16
File Type:pdf, Size:1020Kb
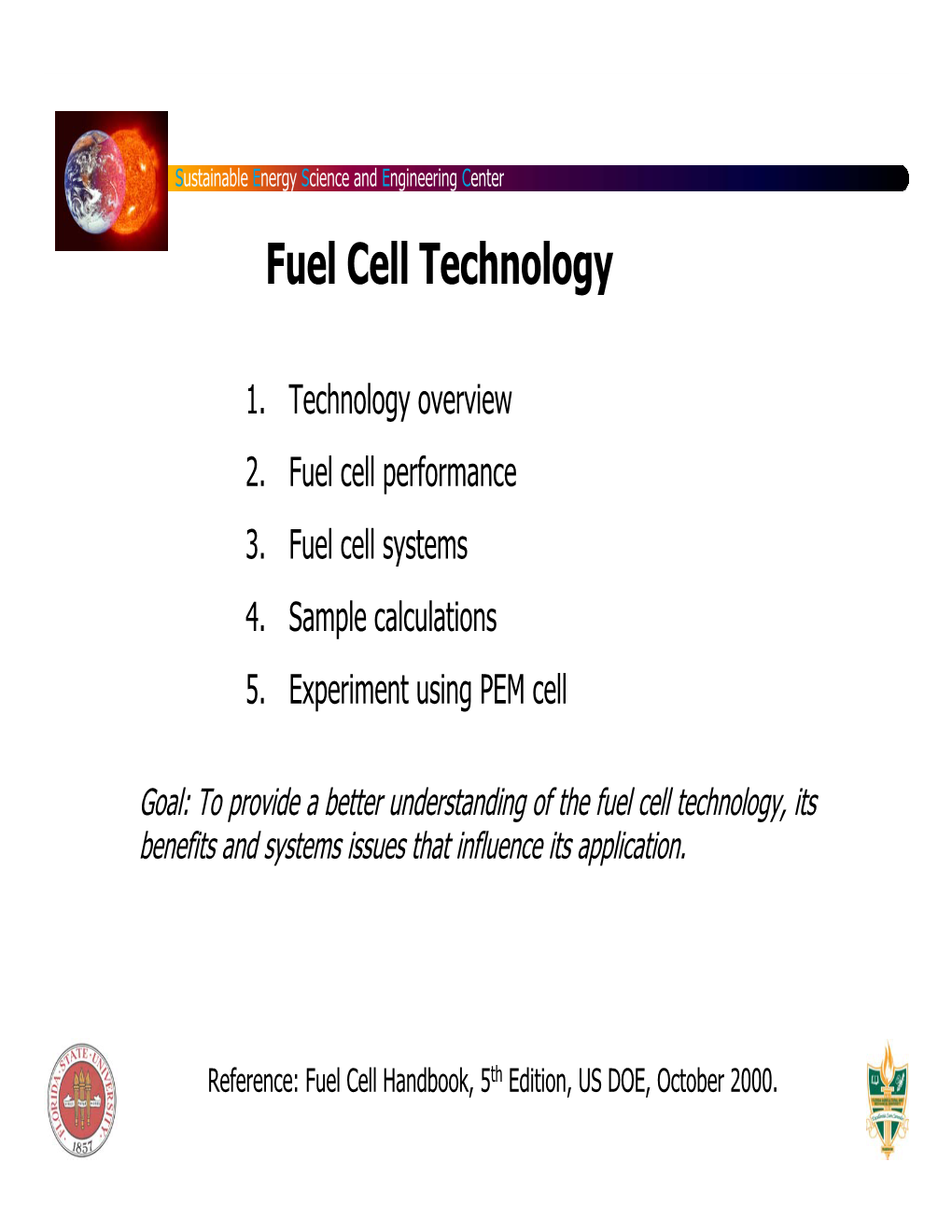
Load more
Recommended publications
-
Section 3.4 Fuel Cells
2016 FUEL CELLS SECTION 3.4 Fuel Cells Fuel cells efficiently convert diverse fuels directly into electricity without combustion, and they are key elements of a broad portfolio for building a competitive, secure, and sustainable clean energy economy. They offer a broad range of benefits, including reduced greenhouse gas emissions; reduced oil consumption; expanded use of renewable power (through the use of hydrogen derived from renewable resources as a transportation fuel as well as for energy storage and transmission); highly efficient energy conversion; fuel flexibility (use of diverse, domestic fuels, including hydrogen, natural gas, biogas, and methanol); reduced air pollution, criteria pollutants, water use; and highly reliable grid support. Fuel cells also have numerous advantages that make them appealing for end users, including quiet operation, low maintenance needs, and high reliability. Because of their broad applicability and diverse uses, fuel cells can address critical challenges in all energy sectors: commercial, residential, industrial, and transportation. The fuel cell industry had revenues of approximately $2.2 billion in 2014, an increase of almost $1 billion over revenues in 2013.1 The largest markets for fuel cells today are in stationary power, portable power, auxiliary power units, backup power, and material handling equipment. Approximately 155,000 fuel cells were shipped worldwide in the four-year period from 2010 through 2013, accounting for 510–583 MW of fuel cell capacity.2 In 2014 alone, more than 50,000 fuel cells accounting for over 180 MW of capacity were shipped.1 In transportation applications, manufacturers have begun to commercialize fuel cell electric vehicles (FCEVs). Hyundai and Toyota have recently introduced their FCEVs in the marketplace, and Honda is set to launch its new FCEV in the market in 2016. -
Iron Based Cathode Catalyst for Alkaline Fuel Cells Thesis Presented in Partial Fulfillment of the Requirements for Honors Resea
Iron Based Cathode Catalyst for Alkaline Fuel Cells Thesis Presented in Partial Fulfillment of the Requirements for Honors Research Distinction at The Ohio State University By Christopher Bruening Undergraduate Program in Chemical Engineering The Ohio State University 2014 Thesis Committee: Dr. Umit Ozkan, Advisor Dr. Kurt Koelling Copyright by Christopher Bruening 2014 Abstract Alkaline fuel cells take advantage of the reaction between hydrogen and oxygen to produce water and an electrical current. During the reaction, a hydroxide ion passes through a membrane from the oxygen side cathode to the hydrogen side anode. Previous research had been conducted on synthesis and evaluation of iron based catalysts for PEM fuel cells by the Heterogeneous Catalysis Research Group at The Ohio State University. PEM fuel cells use a similar reaction between hydrogen and oxygen except a proton travels across the membrane from the hydrogen side to the oxygen side, so the reaction mechanism differs. This paper looks into those same iron based catalysts tested in an alkaline environment. The catalyst in particular is 1% iron in 1:1 Black Pearls 2000 : 1,10 phenanthroline. Those precursors are combined using a wet impregnation and then the result is ball milled. It then undergoes an argon pyrolysis at 1050oC and an ammonia pyrolysis at 950oC which are the final steps in the synthesis. The completed catalyst can then be washed in acid for 1 hour, 2 days, or 1 week to compare the effect of acid washing on different factors. The different catalysts are compared at each stage in the synthesis mainly by their onset potential, which is the voltage where it produces a current density of 0.1 mA/cm2. -
Advanced Ionomers & Meas for Alkaline Membrane Fuel Cells
Advanced Ionomers & MEAs for Alkaline Membrane Fuel Cells PI Bryan Pivovar National Renewable Energy Laboratory June 15, 2018 DOE Hydrogen and Fuel Cells Program 2018 Annual Merit Review and Peer Evaluation Meeting Project ID #FC147 This presentation does not contain any proprietary, confidential, or otherwise restricted information. Overview Timeline and Budget Barriers • Project start: October 2015 • Durability • Project end: March 2019 • Cost • % complete: ~ 70% • Performance • DOE Budget plan Partners – FY 2016 - 2018 $ 2,600k – Cost Share Percentage – 0% • LBNL – Adam Weber • ORNL/UTK – Tom Zawodzinski • Colorado School of Mines – Andy Herring • (in-kind) 3M – Mike Yandrasits NREL | 2 Relevance/Impact DOE (Preliminary) Milestones for AMFCs* • Q2, 2017: Develop anion-exchange membranes with an area specific resistance ≤ 0.1 ohm cm2, maintained for 500 hours during testing at 600 mA/cm2 at T >60 oC. • Q4, 2017: Demonstrate alkaline membrane fuel cell peak power performance > 600 mW/cm2 on 2 H2/O2 (maximum pressure of 1.5 atma) in MEA with a total loading of ≤ 0.125 mgPGM/cm . • Q2, 2019: Demonstrate alkaline membrane fuel cell initial performance of 0.6 V at 600 mA/cm2 on 2 H2/air (maximum pressure of 1.5 atma) in MEA a total loading of < 0.1 mgPGM/cm , and less than 10% voltage degradation over 2,000 hour hold test at 600 mA/cm2 at T>60 oC. Cell may be reconditioned during test to remove recoverable performance losses. • Q2, 2020: Develop non-PGM catalysts demonstrating alkaline membrane fuel cell peak power performance > 600 mW/cm2 under hydrogen/air (maximum pressure of 1.5 atma) in PGM-free MEA. -
Role of Diffuse Layer in Acidic and Alkaline Fuel Cells
Author's personal copy Electrochimica Acta 56 (2011) 4518–4525 Contents lists available at ScienceDirect Electrochimica Acta journal homepage: www.elsevier.com/locate/electacta Role of the diffuse layer in acidic and alkaline fuel cells Isaac Sprague, Prashanta Dutta ∗ School of Mechanical and Materials Engineering, Washington State University, Pullman, WA 99164-2920, USA article info abstract Article history: A numerical model is developed to study electrolyte dependent kinetics in fuel cells. The model is based Received 17 December 2010 on the Poisson–Nernst–Planck (PNP) and generalized-Frumkin–Butler–Volmer (gFBV) equations, and is Received in revised form 13 February 2011 used to understand how the diffuse layer and ionic transport play a role in the performance difference Accepted 15 February 2011 between acidic and alkaline systems. The laminar flow fuel cell (LFFC) is used as the model fuel cell Available online 23 February 2011 architecture to allow for the appropriate comparison of equivalent acidic and alkaline systems. We study the overall cell performance and individual electrode polarizations of acidic and alkaline fuel cells for Keywords: both balanced and unbalanced electrode kinetics as well as in the presence of transport limitations. The Alkaline fuel cell Acidic electrolyte results predict cell behavior based on electrolyte composition that strongly correlates with observed Alkaline electrolyte experimental results from literature and provides insight into the fundamental cause of these results. Electric double layer Specifically, it is found that the working ion concentration at the reaction plane plays a significant role in Laminar flow fuel cell fuel cell performance including activation losses and the response to different kinetic rates at an individual electrode. -
Development of Power Generation by Durect Ethanol Fuel Cell
FUEL CELL SYSTEMS S. Basu New Delhi Department of Chemical Engineering Indian Institute of Technology Delhi INDIA e- Load Water 1. Fuel chamber 2. Oxidant chamber 1 4 2 3. Anode (Pt) 4. Electrolyte Fuel 3 5 Oxidant 5. Cathode (Pt/C) (H ) 2 O2/Air + - H2 2 H +2e (Anode) + - 2 H +1/2 O2 +2e H2O (Cathode) H2 + 1/2 O2 H2O (Overall) 9 Automobile 9 Efficient Power Generation 9 Distributed Power Gen. 9 Environmental Friendly 9 Portable Electronics Eqpt. Fuel Cell Families Phosphoric Acid Cathode Anode o H+ 220 C Solid Polymer Cathode Anode o o H+ 30 C- 80 C Alkaline Air Cathode - Anode Fuel 50 oC- 200 oC OH Molten Carbonates o Cathode = Anode 650 C CO3 Solid Oxides Cathode Anode o o O= 700 C – 1000 C Alkaline Fuel Cell ALKALINE FUEL CELL Alkaline electrolyte - - Anode H2 + 2OH Æ 2H2O + 2e - - Cathode 1/2O2 + H2O + 2e Æ 2OH Overall H2 + 1/2O2 Æ H2O + electrical Energy +heat Poisoning: - 2- CO2 +2OH (CO3) + H2O ¾ Depletion of KOH ¾ Poisoning of cathode surface with carbonates Myth ! 9Kordesch, K. et al., (1999), Intermittent Use of a Low-Cost Alkaline Fuel Cell-hybrid System for Electric Vehicles, J. Power Sources, Vol. 80, pp. 9McLean, G. F. et al. (2002) An assessment of alkaline fuel cell technology, Int. J. Hydrogen Energy 27 507 9Gülzow, E., and Schulze, M. (2003), Long-Term Operation of AFC Electrodes With CO2- Containing Gases, J. Power Sources, Polymer Electrolyte Membrane Fuel Cell (PEMFC) Hydrogen Fuel Cell Direct Alcohol Fuel Cell (DAFC) Fuel Processor Fuel Cleaning System Fuel Pure H2 Electricity Fuel Cell Direct Methanol Fuel Cell Direct Ethanol Fuel Cell (DMFC) (DEFC) Reduce CO adsorption on Pt at High Temp Higher power density at high temperature Easy breakage C-C bond in DEFC at High Temp. -
Relating Catalysis Between Fuel Cell and Metal-Air Batteries
Perspective Relating Catalysis between Fuel Cell and Metal-Air Batteries Matthew Li,1,2 Xuanxuan Bi,1 Rongyue Wang,3 Yingbo Li,4,6 Gaopeng Jiang,2 Liang Li,5 Cheng Zhong,6,* Zhongwei Chen,2,* and Jun Lu1,* With the ever-increasing demand for higher-performing energy-storage sys- Progress and Potential tems, electrocatalysis has become a major topic of interest in an attempt to Catalyst research for fuel cells has enhance the electrochemical performance of many electrochemical technolo- led to much advancement in gies. Discoveries pertaining to the oxygen reduction reaction catalyst helped humanity’s understanding of the enable the commercialization of fuel-cell-based electric vehicles. However, a underlying physics of the process, closely related technology, the metal-air battery, has yet to find commercial significantly enhancing the application. Much like the Li-ion battery, metal-air batteries can potentially uti- performance of the technologies. lize the electrical grid network for charging, bypassing the need for establishing In contrast, metal-air batteries a hydrogen infrastructure. Among the metal-air batteries, Li-air and Zn-air bat- such as Li-air and Zn-air batteries teries have drawn much interest in the past decade. Unfortunately, state-of-the remain to be solved. Although the art metal-air batteries still produce performances that are well below practical metal anode used in this these levels. In this brief perspective, we hope to bridge some of the ideas from systems does play a large role in fuel cell to that of metal-air batteries with the aim of inspiring new ideas and di- limiting their commercial success, rections for future research. -
A Mathematical Model of a Hydrogen/Oxygen Alkaline Fuel Cell Michael C
University of South Carolina Scholar Commons Faculty Publications Chemical Engineering, Department of 1991 A Mathematical Model of a Hydrogen/Oxygen Alkaline Fuel Cell Michael C. Kimble Texas A & M University - College Station Ralph E. White University of South Carolina - Columbia, [email protected] Follow this and additional works at: https://scholarcommons.sc.edu/eche_facpub Part of the Chemical Engineering Commons Publication Info Journal of the Electrochemical Society, 1991, pages 3370-3382. © The Electrochemical Society, Inc. 1991. All rights reserved. Except as provided under U.S. copyright law, this work may not be reproduced, resold, distributed, or modified without the express permission of The Electrochemical Society (ECS). The ra chival version of this work was published in the Journal of the Electrochemical Society. http://www.electrochem.org/ DOI: 10.1149/1.2085416 http://dx.doi.org/10.1149/1.2085416 This Article is brought to you by the Chemical Engineering, Department of at Scholar Commons. It has been accepted for inclusion in Faculty Publications by an authorized administrator of Scholar Commons. For more information, please contact [email protected]. A Mathematical Model of a Hydrogen/Oxygen Alkaline Fuel Cell Michael C. Kimble* and Ralph E. White** Center for Electrochemical Engineering, Department of Chemical Engineering, Texas A&M University, College Station, Texas 77843-3122 ABSTRACT A mathematical model of a hydrogen/oxygen alkaline fuel cell is presented that can be used to predict polarization be- havior under various potential loads. The model describes the phenomena occurring in the solid, liquid, and gaseous phases of the anode, separator, and cathode regions, assuming a macrohomogeneous, three-phase porous electrode struc- ture. -
Cathode Development for Alkaline Fuel Cells Based on a Porous Silver Membrane
*Manuscript text (double-spaced) Click here to view linked References Cathode development for Alkaline Fuel Cells based on a Porous Silver Membrane Abstract: Porous silver membranes were investigated as potential substrates for alkaline fuel cell cathodes by the means of polarisation curves and electrochemical impedance spectroscopy measurements. The silver membranes provide both electrocatalytic function, mechanical support and a means of current collection. Improved performance, compare to a previous design, was obtained by increasing gas accessibility (using Teflon AF instead of PTFE suspension) and by adding a catalyst (MnO 2 or Pt) in the membrane structure to increase the cathode activity. This new cathode design performed significantly better (~ 55 mA cm -2 at 0.8 V, ~295 mA cm -2 at 0.6 V and ~630 mA cm -2 at 0.4 V versus RHE) than the previous design (~ 30 mA cm -2 at 0.8 V, ~250 mA cm -2 at 0.6 V and ~500 mA cm -2 at 0.4 V) in the presence of 6.9 M KOH and oxygen (1 atm(abs)) at room temperature. The hydrophobisation technique of the porous structure and the addition of an extra catalyst appeared to be critical and necessary to obtain high performance. A passive air-breathing hydrogen- air fuel cell constructed from the membranes achieves a peak power density of 65 mW cm -2 at 0.40 V cell potential when operating at 25 oC showing a 15 mW cm -2 improvement compare to the previous design. Keywords: Alkaline fuel cell; gas diffusion electrode; cathode; manganese oxide; silver membrane. -
Alkaline Fuel Cell Engineering and Testing
Technological University Dublin ARROW@TU Dublin Conference Papers School of Mechanical and Design Engineering 2006-01-01 Alkaline Fuel Cell Engineering and Testing James Brunton Technological University Dublin, [email protected] David Kennedy Technological University Dublin, [email protected] Eugene Coyle Technological University Dublin, [email protected] Follow this and additional works at: https://arrow.tudublin.ie/engschmeccon Part of the Mechanical Engineering Commons Recommended Citation Brunton, J., Kennedy, D., Coyle, E.: Alkaline fuel cell engineering and testing. International Conference on Materials, Tribology and Processing, MATRIB, Croatia. 2006. doi:10.21427/f5pe-ak38 This Conference Paper is brought to you for free and open access by the School of Mechanical and Design Engineering at ARROW@TU Dublin. It has been accepted for inclusion in Conference Papers by an authorized administrator of ARROW@TU Dublin. For more information, please contact [email protected], [email protected]. This work is licensed under a Creative Commons Attribution-Noncommercial-Share Alike 4.0 License Alkaline Fuel Cell Engineering and Testing J.Brunton 1, D.M. Kennedy and E.Coyle. Faculty of Engineering Dublin Institute of Technology Dublin 1. [email protected] Abstract A fuel cell is a device that directly converts the chemical energy of reactants (a fuel and an oxidant) into low d. c. electricity [1]. Used in conjunction with other sustainable energy options, hydrogen and fuel cell technology can provide a realistic alternative to fossil fuels. Although research is continuing in many areas we are no closer now to commercialisation than ten to twenty years ago. -
Recent Advances in Application of Chitosan in Fuel Cells Hamideh Vaghari1, Hoda Jafarizadeh-Malmiri1*, Aydin Berenjian2 and Navideh Anarjan3
Vaghari et al. Sustainable Chemical Processes 2013, 1:16 http://www.sustainablechemicalprocesses.com/content/1/1/16 REVIEW Open Access Recent advances in application of chitosan in fuel cells Hamideh Vaghari1, Hoda Jafarizadeh-Malmiri1*, Aydin Berenjian2 and Navideh Anarjan3 Abstract Fuel cells are electrochemical devices which convert chemical energy into electrical energy. Fuel cells have attracted attention due to their potential as a promising alternative to traditional power sources. More recently, efficient and environmentally benign biopolymer “chitosan” have been extensively investigated as a novel material for its application in fuel cells. This biopolymer can be used in both membrane electrolyte and electrode in various fuel cells such as alkaline polymer electrolyte fuel cells, direct methanol fuel cells and biofuel cells. This review provides an overview of main available fuel cells following by application of chitosan as novel biopolymer in fuel cells technology. Recent achievements are included and recommendations are also given for areas of future research. Keywords: Fuel cell, Chitosan, Electrolyte membrane, Electrode, Polymer Introduction polymer electrolytes from renewable sources can become a The extensive use of fossil fuels has resulted to severe promising substitute for synthetic polymers in fuel cells. pollutant emissions, including SOx,NOx, CO, and particu- Among natural polymers, polysaccharides are among the lates which pose severe threat to the health of human be- best candidates due to their abundance in environment [5]. ings [1]. In addition, a steady depletion of world’s limited Chitosan, as a derivative of chitin, is a naturally abundant fossil fuel, reserves calls for efficient, benign and sustainable and low-cost biopolymer which has attracted attention in technologies for energy conversion and power generation. -
Fuel Cell Basics 2012 V2.Key
Introduction to Fuel Cells Scott A Barnett, Northwestern University Motivations, applications, history Fuel cell types Electrochemical Basics Electrochemical Kinetics Charge Transport Mass Transport Stacks and Systems 1 Relevance of Electrochemical Devices: World Energy Flows Sankey Diagram 2 Efficiency Comparisons • High efficiency means low CO2 • Low emissions of other pollutants • Sulfur removed • NOx not formed • No CO • No particulates • Fuel cells can be sited near point of use • Allows direct use of waste heat - effective efficiency > 80% 3 Fuel Cell Introduction • History • Fuel cell issues • Applications • Overview of fuel cell types – Phosphoric acid – Proton exchange membrane – Alkaline – Molten carbonate – Solid oxide 4 Historical Origins of Fuel Cells 5 Fuel Cell Milestones 6 Year of First Successful Operation: Energy Conversion Devices 7 Fuel Cell Problems • Cost – Cost of a new technology is always high initially • Until high-volume manufacturing brings cost down – Need initial applications without entrenched low-cost alternatives • Fuels for fuel cells – Most fuel cells use only pure hydrogen fuel • Not available in large quantities • Difficult to store, transport, transfer, etc. • Usually linked with the “Hydrogen Economy” – Often chemically convert other fuels to hydrogen • Cost – Technology is complex – Fuel cells themselves may be only 25% of total system cost 8 Example Fuel Cell Diagram: Ballard 250 kW System 9 Supplying H2 For Fuel Cells • Steam or dry reforming (methane) ! CH4 + H2O ! CO + 3H2 "H1000K = 206 kJ • -
Prospects for Anion-Exchange Membranes in Alkali Metal–Air Batteries
energies Review Prospects for Anion-Exchange Membranes in Alkali Metal–Air Batteries Misgina Tilahun Tsehaye , Fannie Alloin * and Cristina Iojoiu * Univ. Grenoble Alpes, Univ. Savoie Mont Blanc, CNRS, Grenoble INP, LEPMI, 38 000 Grenoble, France; [email protected] * Correspondence: [email protected] (F.A.); [email protected] (C.I.) Received: 18 October 2019; Accepted: 6 December 2019; Published: 10 December 2019 Abstract: Rechargeable alkali metal–air batteries have enormous potential in energy storage applications due to their high energy densities, low cost, and environmental friendliness. Membrane separators determine the performance and economic viability of these batteries. Usually, porous membrane separators taken from lithium-based batteries are used. Moreover, composite and cation-exchange membranes have been tested. However, crossover of unwanted species (such as zincate ions in zinc–air flow batteries) and/or low hydroxide ions conductivity are major issues to be overcome. On the other hand, state-of-art anion-exchange membranes (AEMs) have been applied to meet the current challenges with regard to rechargeable zinc–air batteries, which have received the most attention among alkali metal–air batteries. The recent advances and remaining challenges of AEMs for these batteries are critically discussed in this review. Correlation between the properties of the AEMs and performance and cyclability of the batteries is discussed. Finally, strategies for overcoming the remaining challenges and future outlooks on the topic are briefly provided. We believe this paper will play a significant role in promoting R&D on developing suitable AEMs with potential applications in alkali metal–air flow batteries.