Investigations of the Isotopic Composition of Trace Gases in the Stratosphere and of the Quantum Dynamics of Non-Adiabatic Atomic Collisions
Total Page:16
File Type:pdf, Size:1020Kb
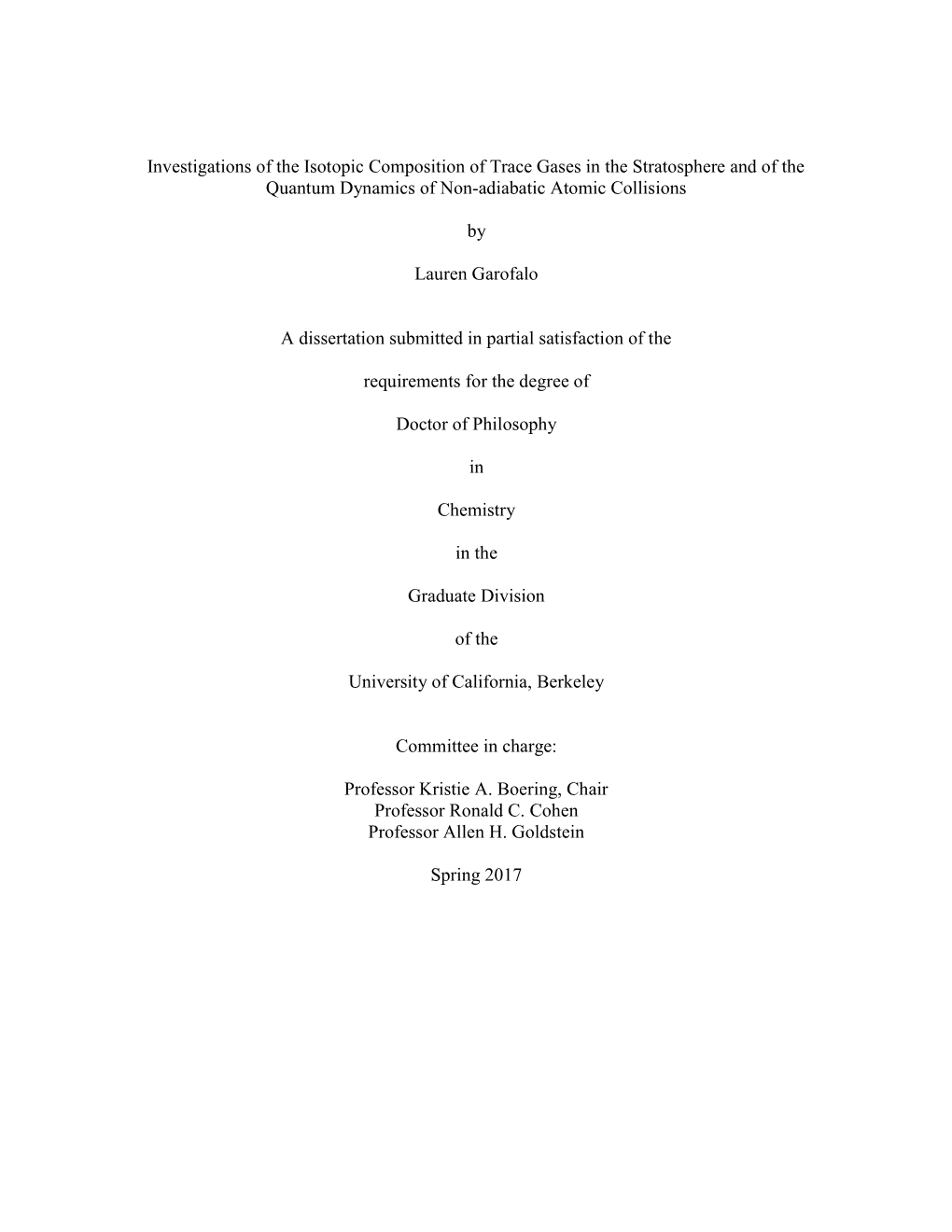
Load more
Recommended publications
-
Walter Alvarez,Professor
DEPARTMENT OF EARTH & PLANETARY SCIENCE DEPARTMENT UPDATE 2017 -2018 FROM THE CHAIR WELCOME TO OUR ANNUAL UPDATE write this introduction after returning from our summer geology field camp. What a treat. I met many of the students when they took their first geoscience class, EPS 50. The capstone field course showed how much these new alumni Ihave grown during their time in EPS to develop into creative and talented geoscientists. On page 13 we hear from one of our 66 new graduates, Departmental Citation recipient Theresa Sawi, about her efforts to understand how earthquakes influence volcanic eruptions. Our faculty, students, staff and alumni continue to make our department one of the world’s best. Our newest faculty member, Bethanie Edwards (page 3), broadens the department’s expertise to include marine biogeochemistry. Along with the arrival of Bill Boos and Daniel Stolper two years ago, we continue to expand our efforts to understand all MARINE SCIENCE aspects of Earth’s changing climate. GET TO KNOW OUR FACULTY There have also been some losses. After 36 years, Tim Teague retired. He may be irreplaceable, but we will try. After three years of dedicated service, always with a smile, Richard Allen’s term as chair ended, and he will continue to direct the Seismological Laboratory. Mark Richards moved to Seattle where he is the University of Washington’s provost. Accolades continue to pour in, and I highlight a few. The American Geophysical Union recognized recent Alumnus Leif BETHANIE EDWARDS Karlstrom with the Kuno Award (page 16) and Professor David Romps with the Atmospheric Sciences Ascent award. -
Near Surface CO2 Triple Oxygen Isotope Composition
Terr. Atmos. Ocean. Sci., Vol. 27, No. 1, 99-106, February 2016 doi: 10.3319/TAO.2015.09.16.01(A) Near Surface CO2 Triple Oxygen Isotope Composition Sasadhar Mahata1, Chung-Ho Wang 2, Sourendra Kumar Bhattacharya1, and Mao-Chang Liang1, 3, 4, 5, * 1 Research Center for Environmental Changes, Academia Sinica, Taipei, Taiwan, R.O.C. 2 Institute of Earth Sciences, Academia Sinica, Taipei, Taiwan, R.O.C. 3 Graduate Institute of Astronomy, National Central University, Taoyuan, Taiwan, R.O.C. 4 Institute of Astronomy and Astrophysics, Academia Sinica, Taipei, Taiwan, R.O.C. 5 Department of Physics, University of Houston, Texas, U.S.A. Received 9 March 2015, revised 14 June 2015, accepted 16 September 2015 ABSTRACT The isotopic composition of carbon dioxide in the atmosphere is a powerful tool for constraining its sources and sinks. In particular, the 17O oxygen anomaly [Δ17O = 1000 × ln(1 + δ17O/1000) - 0.516 × 1000 × ln(1 + δ18O/1000)], with a value > 0.5‰ produced in the middle atmosphere, provides an ideal tool for probing the exchange of carbon dioxide between the biosphere/hydrosphere and atmosphere. The biosphere/hydrosphere and anthropogenic emissions give values ≤ 0.3‰. There- fore, any anomaly in near surface CO2 would reflect the balance between stratospheric input and exchange with the aforemen- 17 tioned surface sources. We have analyzed Δ O values of CO2 separated from air samples collected in Taipei, Taiwan, located in the western Pacific region. The obtained mean anomaly is 0.42 ± 0.14‰ (1-σ standard deviation), in good agreement with model prediction and a published decadal record. -
VOLCANIC ERUPTIONS at PLATE BOUNDARIES Give Your Research the Visibility It Deserves
VOL. 97 NO. 13 1 JUL 2016 Career Paths for African-American Students Did Solar Flares Cook Up Life? High Energy Growth Forecast Through 2040 VOLCANIC ERUPTIONS AT PLATE BOUNDARIES Give Your Research the Visibility it Deserves Abstract Submission Deadline: 3 August Submit by 27 July and Win Big Submit your abstract by 27 July for the chance to win Fall Meeting VIP status, including front row seats for two at all keynote lectures, and more fallmeeting.agu.org Earth & Space Science News Contents 1 JULY 2016 PROJECT UPDATE VOLUME 97, ISSUE 13 9 Creating Career Paths for African-American Students in Geosciences A new initiative at Stony Brook University teaches marketable skills, engages students in research projects, and fosters professional career tracks of underrepresented minorities. NEWS 6 Advisory Panel Calls for Large Increase for Ocean Exploration The recently established Ocean Exploration Advisory Board also urged the National Oceanic and Atmospheric Administration 12 to increase its role in federal coordination of exploration. COVER RESEARCH SPOTLIGHT Understanding Volcanic Eruptions Researchers Attribute Where Plates Meet 31 Human Infl uence on Climate Back to 1930s A new project clarifies the relationships between tectonics and A new study finds that humans likely have volcanic systems and how they influence hazards on Italy’s Mount triggered the past 16 record-breaking hot Etna and Vulcano and Lipari islands. years on Earth, up to 2014. Earth & Space Science News Eos.org // 1 Contents DEPARTMENTS Editor in Chief Barbara T. Richman: AGU, Washington, D. C., USA; eos_ [email protected] Editors Christina M. S. Cohen Wendy S. -
Global 3-D Simulations of the Triple Oxygen Isotope Signature 17O in Atmospheric
RESEARCH ARTICLE Global 3-D Simulations of the Triple Oxygen Isotope 10.1029/2019JD030387 17 Signature Δ O in Atmospheric CO2 Key Points: • This work presents a first view Gerbrand Koren1 , Linda Schneider2,3, Ivar R. van der Velde4,5, Erik van Schaik1, on possible spatial and temporal 6,7 8 8 17 Sergey S. Gromov , Getachew A. Adnew , Dorota J. Mrozek Martino , gradients of Δ OinCO2 across the 8,9 10 11 12 globe Magdalena E. G. Hofmann , Mao-Chang Liang , Sasadhar Mahata , Peter Bergamaschi , • Tropical, boreal, and Southern Ingrid T. van der Laan-Luijkx1, Maarten C. Krol1,8 , Thomas Röckmann8 , 17 Hemisphere observations of Δ Oin and Wouter Peters1,13 CO2 could be of great interest • We implemented spatially and 1Meteorology and Air Quality Group, Wageningen University & Research, Wageningen, The Netherlands, 2Institute of temporally explicit sources and sinks Meteorology and Climate Research (IMK-TRO), Karlsruhe Institute of Technology, Karlsruhe, Germany, 3Now at of Δ17OinCO in a 3-D model 2 4 framework Zentrum für Sonnenenergie- und Wasserstoff-Forschung Baden-Württemberg (ZSW), Stuttgart, Germany, Earth System Research Laboratory, National Oceanic and Atmospheric Administration, Boulder, CO, USA, 5Now at Faculty of Science, VU University Amsterdam, Amsterdam, The Netherlands, 6Atmospheric Chemistry Department, Max-Planck Supporting Information: Institute for Chemistry, Mainz, Germany, 7Institute of Global Climate and Ecology of Roshydromet and RAS, Moscow, • Supporting Information S1 Russia, 8Institute of Marine and Atmospheric Research, Utrecht University, Utrecht, The Netherlands, 9Now at Picarro B.V. 's-Hertogenbosch, The Netherlands, 10Institute of Earth Sciences, Academia Sinica, Taipei, Taiwan, 11Institute of Correspondence to: Global Environmental Change, Xian Jiaotong University, Xian, China, 12European Commission Joint Research Centre, G. -
Drawing Down N2 O
www.unep.org United Nations Environment Programme Drawing Down N2O P. O. Box 30552 - 00100 Nairobi, Kenya Tel.: +254 20 762 1234 Fax: +254 20 762 3927 e-mail: [email protected] To Protect Climate and the Ozone Layer www.unep.org A UNEP Synthesis Report Published by the United Nations Environment Programme (UNEP), November 2013 Copyright © UNEP 2013 ISBN: 978-92-807-3358-7 DEW/1748/NA This publication may be reproduced in whole or in part and in any form for educational or non-profit services without special permission from the copyright holder, provided acknowledgement of the source is made. UNEP would appreciate receiving a copy of any publication that uses this publication as a source. No use of this publication may be made for resale or any other commercial purpose whatsoever without prior permission in writing from the United Nations Environment Programme. Applications for such permission, with a statement of the purpose and extent of the reproduction, should be addressed to the Director, DCPI, UNEP, P. O. Box 30552, Nairobi 00100, Kenya. Disclaimers Mention of a commercial company or product in this document does not imply endorsement by UNEP or the authors. The use of information from this document for publicity or advertising is not permitted. Trademark names and symbols are used in an editorial fashion with no intention on infringement of trademark or copyright laws. We regret any errors or omissions that may have been unwittingly made. © Images and illustrations as specified. Cover Images: All images from Shutterstock. Forest fire: Peter J. Wilson; Agriculture collage: Symbiot; Coal fire plant: Gary Whitton; Atmosphere: Andrew Armyagov. -
Experimental Investigations of Photochemically-Generated Organic Aerosols and Applications to Early Earth and Mars
Experimental investigations of photochemically-generated organic aerosols and applications to early Earth and Mars By Emily Faye Chu A dissertation submitted in partial satisfaction of the requirements for the degree of Doctor of Philosophy in Chemistry in the Graduate Division of the University of California, Berkeley Committee in charge: Professor Kristie A. Boering, Chair Professor Stephen R. Leone Professor Imke de Pater Spring 2013 Abstract Experimental investigations of photochemically-generated organic aerosols and applications to early Earth and Mars By Emily Faye Chu Doctor of Philosophy in Chemistry University of California, Berkeley Professor Kristie A. Boering, Chair Aerosols in planetary atmospheres play a critical role in radiative transfer and thus also in determining the penetration depth of UV radiation and atmospheric temperatures. For early Mars and an anoxic early Earth, aerosols may have significantly influenced the stability of liquid water at the surface, as well as climate and habitability, yet the study of aerosol formation remains poorly constrained by models and experiments, making conclusions difficult. Significant progress can be made in reducing these uncertainties by providing additional laboratory constraints and tests for the photochemical and microphysical models used to generate the greatly varying predictions about aerosol formation in terrestrial-like atmospheres. Photochemistry experiments measuring gas- and condensed-phase species were conducted to determine (1) the extent to which a photochemical -
MILLER INSTITUTE NEWSLETTER Miller Fellow Focus: Alex Thompson
MILLER INSTITUTE NEWSLETTER Fall 2005 Miller Fellow Focus: Alex Thompson Second year Miller Fellow Alex atmospheric non-methane Thompson is an atmospheric chemist hydrocarbons and halocarbons. She working with Prof. Ronald Amundson applied this analysis to very clean air in the Department of Environmental (from the Arctic and the Southern Science, Policy and Management. Ocean), and polluted air (from Toronto and Auckland) in order to How do human activities impact the understand emission patterns and chemistry of the atmosphere? What atmospheric processing, i.e. mixing are the interactions between the and photochemistry. After her Earth’s surface and the atmosphere? doctorate, Alex recognised that the How do these interactions impact the future of global environmental change climate and vice versa? These research involves understanding earth questions are the motivation behind system interfaces, often requiring a Alex’s research. More specifically, multidisciplinary approach. So she Alex is interested in using isotopes to came to UC Berkeley to work with probe the interface between the lower soil scientist and isotope geochemist atmosphere and the oceans, Prof. Ronald Amundson (ESPM). cryosphere, and biosphere. Isotopes They chose to investigate nitrous address the contribution of each of an element have only very small oxide. production pathway using the total differences in their chemical and 15N/14N and 18O/16O content of nitrous physical properties. Their relative Nitrous oxide lasts in the atmosphere oxide or its precursors as a diagnostic. sensitivity or insensitivity to various for a long time (~120 years) and has The problem is that bulk isotope processes allow isotope ratios to be 300 times the global warming signatures are not sufficiently distinct powerful tracers in many areas of potential of CO making it an 2 or well enough defined to differentiate natural science research, including extremely influential greenhouse gas. -
Director National Center for Atmospheric Research
Boulder, Colorado Director National Center for Atmospheric Research Leadership Profile Prepared by Suzanne M. Teer Robert W. Luke July 2018 This leadership profile is intended to provide information about UCAR and the position of Director, National Center for Atmospheric Research. It is designed to assist qualified individuals in assessing their interest in this position. The National Center for Atmospheric Research is a federally funded research and development center sponsored by the National Science Foundation. Since NCAR's founding in 1960, the University Corporation for Atmospheric Research, a nonprofit consortium of 117 North American academic institutions, has managed NCAR on behalf of NSF. The University Corporation for Atmospheric Research is an equal opportunity/equal access/affirmative action employer that strives to develop and maintain a diverse workforce. UCAR is committed to providing equal opportunity for all employees and applicants for employment and does not discriminate on the basis of race, age, creed, color, religion, national origin or ancestry, sex, gender, disability, veteran status, genetic information, sexual orientation, gender identity or expression, or pregnancy. UCAR is committed to inclusivity and promoting an equitable environment that values and respects the uniqueness of all members of the organization. 1 Table of Contents: The Opportunity: Overview 3 The Role: Director of the National Center for Atmospheric Research 4 Qualifications and Personal Qualities 5 Opportunities and Expectations for Leadership 7 The National Center for Atmospheric Research: An Overview 8 The National Science Foundation Cooperative Agreement 11 The University Corporation for Atmospheric Research: An Overview 12 Boulder, Colorado 17 Procedure for Candidacy 19 Appendix I: Diversity Statement 20 Appendix II: NCAR Director Search Committee 22 2 The Opportunity: Overview The University Corporation for Atmospheric Research invites inquiries, nominations, and expressions of interest for the position of Director of the National Center for Atmospheric Research. -
REPORT of the Eighith WMO MEETING of EXPERTS on CARBON DIOXIDE Concenitral"ION and ISOTOPIC MEASUREMENT TECHNIQUES {Edited by 'Thomas Conway)
WORLD METEOROLOGICAL ORGANIZATION GLOBAL ATMOSPHERE WATCH . No. 121 REPORT OF THE EIGHiTH WMO MEETING OF EXPERTS ON CARBON DIOXIDE CONCENiTRAl"ION AND ISOTOPIC MEASUREMENT TECHNIQUES {Edited by 'Thomas Conway) (BOULDER, CO, 6-11 July 1995) . 1' NOTE The designation employed and the presentation of material in this document/publication do not imply the expression of any opinion whatsoever on the part of the Secretariat of the World Meteorological Organization concerning the.legal status of any country, territory, city or area or of its authorities, or concerning the delimitation of its frontiers or boundaries. This report has been compiled from information furnished to the WMO Secretariat. It is not an official WMO publication and its distribution in this form does not imply endorsement by the Organization of the ideas expressed. 1 WORLD METEOROLOGICAL ORGANIZATION ~;~~~ GLOBAL ATMOSPHERE WATCH S::1-1~~-~=- 0/-·-·6t2 f No. 121 , ., / J ~ · - ~ REPORT OF THE EIGHTH WMO MEETING OF EXPERTS ON CARBON DIOXIDE CONCENTRATION AND ISOTOPIC MEASUREMENT TECHNIQUES {Edited by Thomas Conway) (BOULDER, CO, 6-11July1995) WMO-T D No. 821 TABLE OF CONTENTS 1. OPENING OF THE MEETING . .. ................................... .. .. .. .. .. .. .. .. .. .. .. ... .. .. .. .. .. .. .. .. 1 2. INTERCOMPARISON OF FLASK AND IN SITU C02 PROGRAMMES AT ALERT, NORTHWEST TERRITORIES..................................................................... 1 2.1 Conditions for Study............................................. ..................................................... -
Chemical Removal of Nitrogen Oxides from the Atmosphere: Impacts on Air Quality and Effects of Temperature
Chemical removal of nitrogen oxides from the atmosphere: Impacts on air quality and effects of temperature by Paul Romer A dissertation submitted in partial satisfaction of the requirements for the degree of Doctor of Philosophy in Chemistry in the Graduate Division of the University of California, Berkeley Committee in charge: Professor Ronald C. Cohen, Chair Professor Kristie A. Boering Professor Allen H. Goldstein Summer 2018 Chemical removal of nitrogen oxides from the atmosphere: Impacts on air quality and effects of temperature Copyright 2018 by Paul Romer 1 Abstract Chemical removal of nitrogen oxides from the atmosphere: Impacts on air quality and effects of temperature by Paul Romer Doctor of Philosophy in Chemistry University of California, Berkeley Professor Ronald C. Cohen, Chair The concentration of nitrogen oxides (NOx ≡ NO + NO2) regulates the concentrations of all major atmospheric oxidants and the formation of secondary air pollutants, with conse- quences for climate, human health, and ecosystems. The chemical cycling between NOx and its oxidation products controls the concentration and transport of NOx and affects the spatial extent of air pollution. Previous studies have shown that permanent loss of NOx occurs both through production of HNO3 and through production of alkyl and multifunctional nitrates (RONO2). Despite their importance to atmospheric chemistry, significant uncertainties re- main in the relative importance of these pathways and in the fates of RONO2 and HNO3 in the atmosphere. I use observations from two intensive field studies, the Southern Oxidant and Aerosol Study (SOAS) in Centreville Alabama and the Korea-United States Air Quality Study (KORUS-AQ) over South Korea, to provide new constraints on the lifetimes and fates of NOx oxidation products. -
UC Berkeley UC Berkeley Electronic Theses and Dissertations
UC Berkeley UC Berkeley Electronic Theses and Dissertations Title Observing the Chemistry of Cities: Space-based Spectroscopy of NO2 Permalink https://escholarship.org/uc/item/70p0x81q Author Valin, Lukas Carl Publication Date 2012 Peer reviewed|Thesis/dissertation eScholarship.org Powered by the California Digital Library University of California Observing the Chemistry of Cities: Space-based Spectroscopy of NO 2 By Lukas C. Valin A dissertation submitted in partial satisfaction of the requirements for the degree of Doctor of Philosophy In Chemistry in the Graduate Division of the University of California, Berkeley Committee in charge: Professor Ronald C. Cohen, Chair Professor Kristie A. Boering Professor Inez Fung Fall 2012 Abstract Observing the Chemistry of Cities: Space-based Spectroscopy of NO 2 By Lukas C. Valin Doctor of Philosophy in Chemistry University of California, Berkeley Professor Ronald Cohen, Chair The atmospheric chemistry of cities depends strongly on the concentration of the hydroxyl radical (OH). OH removes organic molecules ( τ = minutes – days), nitrogen oxides (NO x: NO + NO 2; τ = 2 – 20 h), and sulfur dioxide (τ = 2 – 3 d) from the atmosphere and forms ozone and particulate matter in the process. The spatial and temporal variability of OH is not fully understood due in large part to its strong nonlinear dependence on the concentration of NO x. In this dissertation, I use measurements of the NO 2 column from the Ozone Monitoring Instrument (OMI), a satellite-based UV/Visible spectrometer, and calculations from chemical transport models to investigate the relationship of OH and NOx in urban regions. I characterize the instrumental and model performance necessary to accurately infer OH concentration from space- based measurements of the NO 2 column. -
Nitrous Oxide and Molecular Nitrogen Isotopic Compositions and Aerosol Optical Properties: Experiments and Observations Relevant to Planetary Atmospheres
Nitrous Oxide and Molecular Nitrogen Isotopic Compositions and Aerosol Optical Properties: Experiments and Observations Relevant to Planetary Atmospheres by Philip Louis Croteau A dissertation submitted in partial satisfaction of the requirements for the degree of Doctor of Philosophy in Chemistry in the Graduate Division of the University of California, Berkeley Committee in charge: Professor Kristie A. Boering, Chair Professor Ronald C. Cohen Professor Imke de Pater Spring 2010 Nitrous Oxide and Molecular Nitrogen Isotopic Compositions and Aerosol Optical Properties: Experiments and Observations Relevant to Planetary Atmospheres © 2010 by Philip Louis Croteau Abstract Nitrous Oxide and Molecular Nitrogen Isotopic Compositions and Aerosol Optical Properties: Experiments and Observations Relevant to Planetary Atmospheres by Philip Louis Croteau Doctor of Philosophy in Chemistry University of California, Berkeley Professor Kristie A. Boering, Chair Nitrous oxide (N 2O) and molecular nitrogen (N 2) isotopic compositions and aerosol optical properties were investigated through experiments and observations to elucidate their roles in atmospheric radiative transfer and chemistry. In Earth’s atmosphere, the isotopic composition of N2O, a potent greenhouse gas, is a useful tool for investigating its sources and sinks. N 2 is the main component of the atmospheres of Earth and Titan, and isotope effects in its photoionization may lead to isotopic fractionation. The optical properties of aerosols, a component of most planetary atmospheres, determine