Electrochemical Surface Modification of a 2D Mos2 Semiconductor
Total Page:16
File Type:pdf, Size:1020Kb
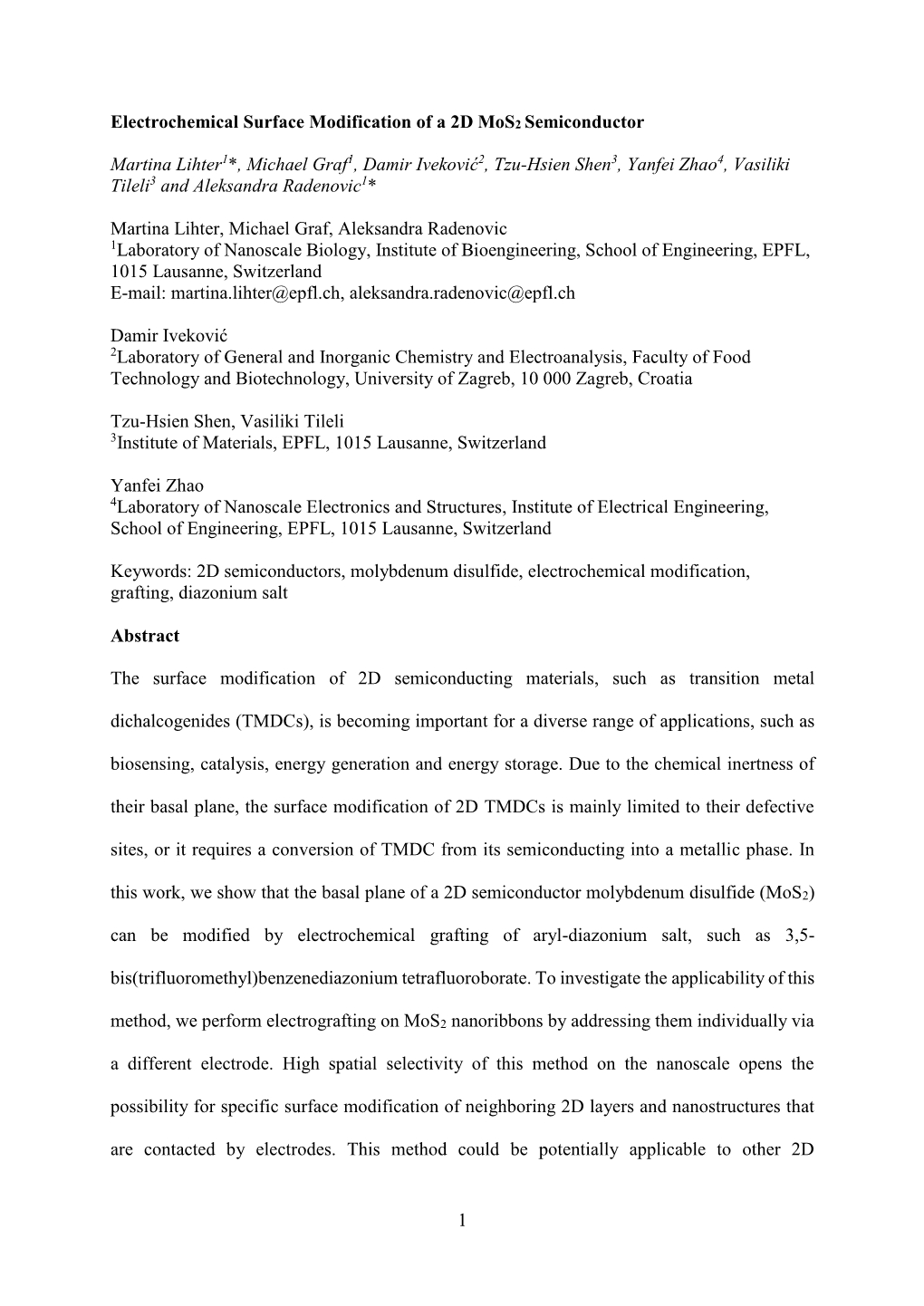
Load more
Recommended publications
-
Electrochemical Reaction in Single Layer Mos2: Nanopores Opened Atom by Atom
Electrochemical reaction in single layer MoS2: nanopores opened atom by atom J. Feng1, K. Liu1, M.Graf1, M.Lihter1,2, R. D. Bulushev1, D. Dumcenco3, D.T.L. Alexander4, D. Krasnozhon3, T. Vuletic2, A.Kis3, and A.Radenovic1 1Laboratory of Nanoscale Biology, Institute of Bioengineering, School of Engineering, EPFL, 1015 Lausanne, Switzerland 2Institut za fiziku, Bijenička 46,Zagreb, Croatia 3 Laboratory of Nanoscale Electronics and Structure, Institute of Electrical Engineering , School of Engineering, EPFL, 1015 Lausanne, Switzerland 4 Centre Interdisciplinaire de Microscopie Électronique (CIME), EPFL, 1015 Lausanne, Switzerland *Correspondence should be addressed [email protected] and [email protected] ABSTRACT Ultrathin nanopore membranes based on 2D materials have demonstrated ultimate resolution toward DNA sequencing. Among them, molybdenum disulphide (MoS2) shows long-term stability as well as superior sensitivity enabling high throughput performance. The traditional method of fabricating nanopores with nanometer precision is based on the use of focused electron beams in transmission electron microscope (TEM). This nanopore fabrication process is time-consuming, expensive, not scalable and hard to control below 1 nm. Here, we exploited the electrochemical activity of MoS2 and developed a convenient and scalable method to controllably make nanopores in single-layer MoS2 with sub-nanometer precision using electrochemical reaction (ECR). The electrochemical reaction on the surface of single-layer MoS2 is initiated at the 1 location of defects or single atom vacancy, followed by the successive removals of individual atoms or unit cells from single-layer MoS2 lattice and finally formation of a nanopore. Step-like features in the ionic current through the growing nanopore provide direct feedback on the nanopore size inferred from a widely used conductance vs. -
Detection of RNAP-DNA Complexes Using Solid State Nanopores Camille Raillon, Pierre Granjon, Michael Graf, Aleksandra Radenovic
Detection of RNAP-DNA complexes using solid state nanopores Camille Raillon, Pierre Granjon, Michael Graf, Aleksandra Radenovic To cite this version: Camille Raillon, Pierre Granjon, Michael Graf, Aleksandra Radenovic. Detection of RNAP-DNA complexes using solid state nanopores. EMBC 2013 - 35th Annual International Conference of the IEEE Engineering in Medicine and Biology Society, Jul 2013, Osaka, Japan. pp.4106-4109, 10.1109/EMBC.2013.6610448. hal-00872610 HAL Id: hal-00872610 https://hal.archives-ouvertes.fr/hal-00872610 Submitted on 14 Jan 2015 HAL is a multi-disciplinary open access L’archive ouverte pluridisciplinaire HAL, est archive for the deposit and dissemination of sci- destinée au dépôt et à la diffusion de documents entific research documents, whether they are pub- scientifiques de niveau recherche, publiés ou non, lished or not. The documents may come from émanant des établissements d’enseignement et de teaching and research institutions in France or recherche français ou étrangers, des laboratoires abroad, or from public or private research centers. publics ou privés. 35th Annual International Conference of the IEEE EMBS Osaka, Japan, 3 - 7 July, 2013 Detection of RNAP-DNA complexes using solid state nanopores C. Raillon, P. Granjon, M. Graf and A. Radenovic Abstract—Transcription is the first step in gene expression show how nanopore sensing and nanopore based force where DNA is copied into RNA. It is extensively studied at spectroscopy can be used to study RNAP-DNA interaction at the bulk level especially the regulation mechanism, which in the single-molecule level. cancerous cells is impaired. We were interested in studying E. coli RNAP enzyme at the single-molecule level for its functional as well as molecular motor properties. -
Measurement of the Position-Dependent Electrophoretic Force on DNA in a Glass Nanocapillary † † † ‡ § Roman D
Letter pubs.acs.org/NanoLett Measurement of the Position-Dependent Electrophoretic Force on DNA in a Glass Nanocapillary † † † ‡ § Roman D. Bulushev, Lorenz J. Steinbock, Sergey Khlybov, Julian F. Steinbock, Ulrich F. Keyser, † and Aleksandra Radenovic*, † Laboratory of Nanoscale Biology, Institute of Bioengineering, School of Engineering, EPFL, 1015 Lausanne, Switzerland ‡ Faculty of Physics, Technical University of Kaiserslautern, 67663 Kaiserslautern, Germany § Cavendish Laboratory, University of Cambridge, Cambridge CB3 0HE, United Kingdom *S Supporting Information ABSTRACT: The electrophoretic force on a single DNA molecule inside a glass nanocapillary depends on the opening size and varies with the distance along the symmetrical axis of the nanocapillary. Using optical tweezers and DNA-coated beads, we measured the stalling forces and mapped the position-dependent force profiles acting on DNA inside nanocapillaries of different sizes. We showed that the stalling force is higher in nanocapillaries of smaller diameters. The position-dependent force profiles strongly depend on the size of the nanocapillary opening, and for openings smaller than 20 nm, the profiles resemble the behavior observed in solid-state nanopores. To characterize the position-dependent force profiles in nanocapillaries of different sizes, we used a model that combines information from both analytical approximations and numerical calculations. KEYWORDS: Single molecule measurements, nanocapillary, solid-state nanopore, DNA translocation, optical tweezers, force measurements olid-state nanopores are label-free sensing platforms for the ultrasensitive force measurement experiments.17,18 In this S detailed characterization of single molecules. They have combination, nanocapillaries have advantages over nanopores found applications as detectors of DNA,1 RNA,2 proteins,3 and due to a simpler design of the microfluidic cell, more sensitive DNA−protein complexes4 and are currently promising lateral displacement of the optically trapped bead during DNA 5,6 candidates for next generation DNA sequencing. -
Single Molecule Localization and Discrimination of DNA−Protein Complexes by Controlled Translocation Through Nanocapillaries Roman D
Letter pubs.acs.org/NanoLett Single Molecule Localization and Discrimination of DNA−Protein Complexes by Controlled Translocation Through Nanocapillaries Roman D. Bulushev,† Sanjin Marion,*,‡ Ekaterina Petrova,§ Sebastian J. Davis,† Sebastian J. Maerkl,§ and Aleksandra Radenovic† † Laboratory of Nanoscale Biology, Institute of Bioengineering, School of Engineering, EPFL, 1015 Lausanne, Switzerland ‡ Institute of Physics, Bijenickǎ cesta 46, HR-10000 Zagreb, Croatia § Laboratory of Biological Network Characterization, Institute of Bioengineering, School of Engineering, EPFL, 1015 Lausanne, Switzerland *S Supporting Information ABSTRACT: Through the use of optical tweezers we performed controlled translocations of DNA−protein com- plexes through nanocapillaries. We used RNA polymerase (RNAP) with two binding sites on a 7.2 kbp DNA fragment and a dCas9 protein tailored to have five binding sites on λ-DNA (48.5 kbp). Measured localization of binding sites showed a shift from the expected positions on the DNA that we explained using both analytical fitting and a stochastic model. From the measured force versus stage curves we extracted the non- equilibrium work done during the translocation of a DNA−protein complex and used it to obtain an estimate of the effective charge of the complex. In combination with conductivity measurements, we provided a proof of concept for discrimination between different DNA−protein complexes simultaneous to the localization of their binding sites. KEYWORDS: Nanopore, nanocapillary, force measurements, DNA−protein complex, protein binding site, Jarzynski equation NA−protein interactions ubiquitously regulate almost all protein conformation and as a result the proper DNA−protein D aspects of cellular function, such as DNA chromosome interaction. maintenance, replication, transcriptional regulation, and DNA Single-molecule (SM) techniques have emerged to comple- repair.1 Most of these interactions occur after complex protein ment these methods and are suitable to characterize rare search and binding to a sequence specific DNA target. -
High-Throughput and Adaptive Super-Resolution Fluorescence Microscopy of Organelles
Thèse n° 10 326 High-throughput and adaptive super-resolution fluorescence microscopy of organelles Présentée le 17 septembre 2020 à la Faculté des sciences de base Laboratoire de biophysique expérimentale Programme doctoral en photonique pour l’obtention du grade de Docteur ès Sciences par Dora MAHECIC Acceptée sur proposition du jury Prof. A. Radenovic, présidente du jury Prof. S. Manley, directrice de thèse Dr U. Manor, rapporteur Prof. B. Huang, rapporteur Dr M. Weigert, rapporteur 2020 Acknowledgements Acknowledgements First, I would like to thank my thesis supervisor and mentor Prof. Suliana Manley for giving me the opportunity to be a part of her lab, meet amazing scientists and work on fascinating projects. I have learned so much over the past 4 years, and the skills and experience I have gathered here will follow me throughout my future career, wherever that might be. I would also like to thank the members of my thesis defense jury Prof. Bo Huang, Dr. Uri Manor, Prof. Aleksandra Radenovic and Dr. Martin Weigert, for their time and valuable feedback. While I am saddened to not have met all of you in person, I am hopeful that our paths will cross in the near future! Next, thank you to all the past and present lab members for enriching my every workday with stimulating discussions. More specifically, a big thank you to Anna Archetti, Isabelle Coke, Gaby Correa, Kyle Douglass, Davide Gambarotto, Juliette Griffié, Khalid Ibrahim, Tatjana Kleele, Ambroise Lambert, Sofia Magkiriadou, Hélène Perreten Lambert, Timo Rey, Christian Sieben, Jenny Sülzle, Aster Vanhecke, Quentin Wannebroucq, Julius Winter, Sofia Zaganelli, Chen Zhang. -
13Th International School of Biophysics: ABC of Physics of Life
1133tthh IInntteerrnnaattiioonnaall SScchhooooll ooff BBiioopphhyyssiiccss ABCABC ofof PhysicsPhysics ofof LifeLife BOOKBOOK OFOF ABSTRACTSABSTRACTS CCrrooaattiiaa,, SSeepptteemmbbeerr 0011--1100,, 22001166.. BOOK OF ABSTRACTS ABC of Physics of Life The 13th International "Greta Pifat Mrzljak" School of Biophysics "ABC of Physics of Life: Book of abstracts" Adriatic Coast, Croatia/ 1st– 10th September Published by: Croatian Biophysical Society & Institute of Physics, Zagreb, Croatia Editors: Sanjin Marion, Ida Delač Marion, Nadica Maltar Strmečki, Sanja Josef-Golubić and Tomislav Vuletić ISBN: 978-953-7666-14-9 Circulation: 130 copies Book of abstracts FOREWORD The School This session of the International School of Biophysics is the thirteenth in a series held for 35 years across Croatia: in Dubrovnik, Rovinj and Primošten. The previous sessions were held in 1981, 1984, 1987, 1990, 1994, 1997, 2000, 2003, 2006, 2009, 2012 and 2014. In the period 1981-2009, the school was chaired by the late Prof. Greta Pifat-Mrzljak, an eminent Croatian biophysicist, president of Croatian Biophysical Society and a member of the International Union for Pure and Applied Biophysics (IUPAB) Council. She was also awarded the American Biophysical Society’s Emily M. Gray Award, primarily for her inspiring achievement with the School. After passing of prof. Pifat Mrzljak in 2009, it was widely agreed that this school of biophysics for young scientists is still needed. In 2012 the Croatian Biophysical Society and Ruđer Bošković Institute have committed their resources in order to assure the future of the school and a new School was organized as a collective undertaking. Since then, we have had a strong support by EBSA (European Biophysical Societies’ Association) and the Lecturers themselves, who commit their time and financial resources as a greatest sign of the need for the School to keep going. -
Fast and Automatic Processing of Multi-Level Events in Nanopore
Fast and automatic processing of multi-level events in nanopore translocation experiments Camille Raillon, Pierre Granjon, Michael Graf, Lorentz Steinbock, Aleksandra Radenovic To cite this version: Camille Raillon, Pierre Granjon, Michael Graf, Lorentz Steinbock, Aleksandra Radenovic. Fast and automatic processing of multi-level events in nanopore translocation experiments. Nanoscale, Royal Society of Chemistry, 2012, 14 (16), pp.4916-4924. 10.1039/C2NR30951C. hal-00717284 HAL Id: hal-00717284 https://hal.archives-ouvertes.fr/hal-00717284 Submitted on 22 Aug 2013 HAL is a multi-disciplinary open access L’archive ouverte pluridisciplinaire HAL, est archive for the deposit and dissemination of sci- destinée au dépôt et à la diffusion de documents entific research documents, whether they are pub- scientifiques de niveau recherche, publiés ou non, lished or not. The documents may come from émanant des établissements d’enseignement et de teaching and research institutions in France or recherche français ou étrangers, des laboratoires abroad, or from public or private research centers. publics ou privés. View Online / Journal Homepage Nanoscale Dynamic Article LinksC< Cite this: DOI: 10.1039/c2nr30951c www.rsc.org/nanoscale FEATURE ARTICLE Fast and automatic processing of multi-level events in nanopore translocation experiments† C. Raillon,a P. Granjon,b M. Graf,a L. J. Steinbocka and A. Radenovic*a Received 20th April 2012, Accepted 13th June 2012 DOI: 10.1039/c2nr30951c We have developed a method to analyze in detail, translocation events providing a novel and flexible tool for data analysis of nanopore experiments. Our program, called OpenNanopore, is based on the cumulative sums algorithm (CUSUM algorithm). This algorithm is an abrupt change detection algorithm that provides fitting of current blockages, allowing the user to easily identify the different levels in each event. -
Aleksandra Radenovic Marco Scarselli, Paolo Annibale And
Membrane Biology: Cell Type-specific β2-Adrenergic Receptor Clusters Identified Using Photoactivated Localization Microscopy Are Not Lipid Raft Related, but Depend on Actin Cytoskeleton Integrity Downloaded from Marco Scarselli, Paolo Annibale and Aleksandra Radenovic J. Biol. Chem. 2012, 287:16768-16780. doi: 10.1074/jbc.M111.329912 originally published online March 22, 2012 http://www.jbc.org/ Access the most updated version of this article at doi: 10.1074/jbc.M111.329912 Find articles, minireviews, Reflections and Classics on similar topics on the JBC Affinity Sites. at EPFL Scientific Information and Libraries on July 20, 2014 Alerts: • When this article is cited • When a correction for this article is posted Click here to choose from all of JBC's e-mail alerts Supplemental material: http://www.jbc.org/jbc/suppl/2012/03/22/M111.329912.DC1.html This article cites 47 references, 17 of which can be accessed free at http://www.jbc.org/content/287/20/16768.full.html#ref-list-1 THE JOURNAL OF BIOLOGICAL CHEMISTRY VOL. 287, NO. 20, pp. 16768–16780, May 11, 2012 © 2012 by The American Society for Biochemistry and Molecular Biology, Inc. Published in the U.S.A. Cell Type-specific 2-Adrenergic Receptor Clusters Identified Using Photoactivated Localization Microscopy Are Not Lipid Raft Related, but Depend on Actin Cytoskeleton Integrity*□S Received for publication, December 3, 2011, and in revised form, March 14, 2012 Published, JBC Papers in Press, March 22, 2012, DOI 10.1074/jbc.M111.329912 Marco Scarselli1, Paolo Annibale1, and Aleksandra Radenovic2 From the Laboratory of Nanoscale Biology, Institute of Bioengineering, School of Engineering, E´cole Polytechnique Fe´de´rale de Lausanne (EPFL), CH-1015 Lausanne, Switzerland Background: The direct measurement of diffraction-limited structures, such as clusters, is outside the resolution of the available microscopy techniques. -
Electrochemical Reaction in Single Layer Mos2: Nanopores Opened Atom by Atom † † † † ‡ † § ∥ J
Letter pubs.acs.org/NanoLett Electrochemical Reaction in Single Layer MoS2: Nanopores Opened Atom by Atom † † † † ‡ † § ∥ J. Feng, K. Liu,*, M. Graf, M. Lihter, , R. D. Bulushev, D. Dumcenco, D. T. L. Alexander, § ‡ § † D. Krasnozhon, T. Vuletic, A. Kis, and A. Radenovic*, † Laboratory of Nanoscale Biology, Institute of Bioengineering, School of Engineering, EPFL, 1015 Lausanne, Switzerland ‡ Institut za fiziku, Bijenickǎ 46, Zagreb, Croatia § Laboratory of Nanoscale Electronics and Structure, Institute of Electrical Engineering, School of Engineering, EPFL, 1015 Lausanne, Switzerland ∥ Centre Interdisciplinaire de Microscopie Électronique (CIME), EPFL, 1015 Lausanne, Switzerland *S Supporting Information ABSTRACT: Ultrathin nanopore membranes based on 2D materials have demonstrated ultimate resolution toward DNA sequencing. Among them, fi molybdenum disul de (MoS2) shows long-term stability as well as superior sensitivity enabling high throughput performance. The traditional method of fabricating nanopores with nanometer precision is based on the use of focused electron beams in transmission electron microscope (TEM). This nanopore fabrication process is time-consuming, expensive, not scalable, and hard to control below 1 nm. Here, we exploited the electrochemical activity of MoS2 and developed a convenient and scalable method to controllably make nanopores in single-layer MoS2 with subnanometer precision using electrochemical reaction (ECR). The electrochemical reaction on the surface of single-layer MoS2 is initiated at the location of defects or single atom vacancy, followed by the successive removals of individual atoms or unit cells from single-layer MoS2 lattice and finally formation of a nanopore. Step-like features in the ionic current through the growing nanopore provide direct feedback on the nanopore size inferred from a widely used conductance vs pore size model. -
Nanocapillaries Combined with Optical Tweezers As a Single Molecule Technique for Studying DNA-Protein Complexes
Nanocapillaries combined with optical tweezers as a single molecule technique for studying DNA-protein complexes THÈSE NO 7608 (2017) PRÉSENTÉE LE 7 AVRIL 2017 À LA FACULTÉ DES SCIENCES ET TECHNIQUES DE L'INGÉNIEUR LABORATOIRE DE BIOLOGIE À L'ÉCHELLE NANOMÉTRIQUE PROGRAMME DOCTORAL EN BIOTECHNOLOGIE ET GÉNIE BIOLOGIQUE ÉCOLE POLYTECHNIQUE FÉDÉRALE DE LAUSANNE POUR L'OBTENTION DU GRADE DE DOCTEUR ÈS SCIENCES PAR Roman BULUSHEV acceptée sur proposition du jury: Prof. Ph. Renaud, président du jury Prof. A. Radenovic, directrice de thèse Prof. U. Keyser, rapporteur Prof. R. Lim, rapporteur Prof. S. Maerkl, rapporteur Suisse 2017 "Unless you try to do something beyond what you have already mastered, you will never grow." — Ronald E. Osborn Abstract Interactions of proteins with DNA are essential for carrying out DNA’s biological functions and performing a cellular cycle. Such processes as DNA replication, expression and repair are performed by an organised action of various proteins. To better understand the function of protein machinery many methods have been developed over the years. They can be divided into two categories: single molecule and bulk techniques. In comparison to bulk experiments, where the effect of an ensemble of proteins is measured, single molecule techniques analyse each molecule one by one. This fact allows to detect rare events and avoid averaging over the population. Moreover, some single molecule techniques can be used for measuring dynamics and active processes of biomolecules. The objective of this thesis was to make a single molecule technique combining nanocapillaries and optical tweezers for characterisation of DNA-protein complexes. There were three main steps in this thesis: 1) building and characterisation of the setup 2) using it for detection and characterisation of DNA-protein complexes and 3) localisation and discrimination of DNA-protein complexes. -
Controlling DNA Capture and Propagation
NANO LETTERS 2007 Controlling DNA Capture and Vol. 7, No. 9 Propagation through Artificial 2824-2830 Nanopores Eliane H. Trepagnier,†,‡,§,| Aleksandra Radenovic,†,§,| David Sivak,‡,⊥ Phillip Geissler,‡,⊥ and Jan Liphardt*,‡,§,| Biophysics Graduate Group, UniVersity of California, Berkeley, Berkeley, California, Department of Physics, UniVersity of California, Berkeley, Berkeley, California, Physical Biosciences DiVision, Lawrence Berkeley National Laboratory (LBL), Berkeley, California, and College of Chemistry, UniVersity of California, Berkeley, Berkeley, California Received June 15, 2007; Revised Manuscript Received July 30, 2007 ABSTRACT Electrophorescing biopolymers across nanopores modulates the ionic current through the pore, revealing the polymer’s diameter, length, and conformation. The rapidity of polymer translocation (∼30 000 bp/ms) in this geometry greatly limits the information that can be obtained for each base. Here we show that the translocation speed of λ-DNA through artificial nanopores can be reduced using optical tweezers. DNAs coupled to optically trapped beads were presented to nanopores. DNAs initially placed up to several micrometers from the pore could be captured. Subsequently, the optical tweezers reduced translocation speeds to 150 bp/ms, about 200-fold slower than free DNA. Moreover, the optical tweezers allowed us to “floss” single polymers back and forth through the pore. The combination of controlled sample presentation, greatly slowed translocation speeds, and repeated electrophoresis of single DNAs removes several barriers to using artificial nanopores for sequencing, haplotyping, and characterization of protein−DNA interactions. The characterization of single biopolymers is one of the demonstrated by Keyser et al.12 These authors used optical potential applications of artificial nanopores1,2 and nano- force measurement to calculate the electrical force exerted - channels.3 5 For example, artificial nanopores have been on a DNA molecule in a nanopore as well as the effective from https://pubs.acs.org/doi/10.1021/nl0714334. -
Pressure Induced Enlargement and Ionic Current Rectification In
Pressure Induced Enlargement and Ionic Current Rectification in Symmetric Nanopores Sebastian J. Davis,1 Michal Macha,1 Andrey Chernev,1 David M. Huang,2 Aleksandra Radenovic,1, ∗ and Sanjin Marion1, ∗ 1Laboratory of Nanoscale Biology, Institute of Bioengineering, School of Engineering, EPFL, 1015 Lausanne, Switzerland 2Department of Chemistry, School of Physical Sciences, The University of Adelaide, Adelaide, Australia Nanopores in solid state membranes are a tool able to probe nanofluidic phenomena, or can act as a single molecular sensor. They also have diverse applications in filtration, desalination, or osmotic power generation. Many of these applications involve chemical, or hydrostatic pressure differences which act on both the supporting membrane, and the ion transport through the pore. By using pressure differences between the sides of the membrane, and an alternating current approach to probe ion transport, we investigate two distinct physical phenomena: the elastic deformation of the membrane through the measurement of strain at the nanopore, and the growth of ionic current rectification with pressure due to pore entrance effects. I. INTRODUCTION translocations.24,25 No experiments have been performed so far with nanopores in elastic solid-state membranes, Nanopores are a single molecule tool with diverse ap- although such membranes are usually used in condi- plications in bio-sensing,1,2 osmotic power generation3 tions of osmotic or hydraulic pressure gradients which and water desalination.4 A nanoscale pore separates two could influence pore properties like ion selectivity and 3,4,26 reservoirs filled with electrolyte. Monitoring ion trans- water permeability. Theoretical work on sub-nm port through the pore yields information about a passing pores in 2D materials indicates the presence of strong 27{31 analyte such as DNA, or on non-linear phenomena such mechanosensitivity to lateral stresses.