Antidunes on Steep Slopes A
Total Page:16
File Type:pdf, Size:1020Kb
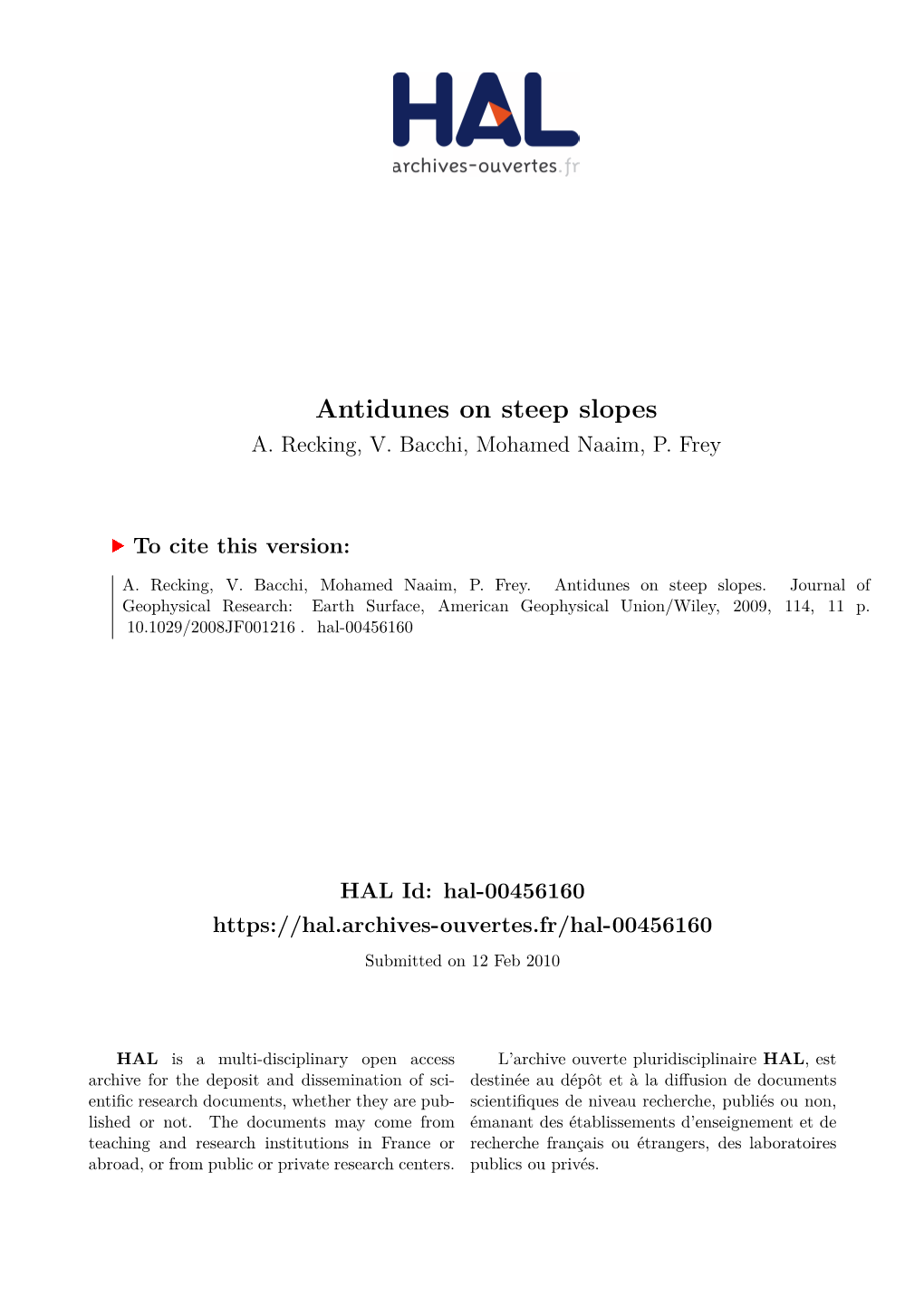
Load more
Recommended publications
-
The Depositional Signature of Strongly Aggradational Chute-And-Pool Bedforms
Marine and River Dune Dynamics – MARID VI – 1-3 April 2019 - Bremen, Germany Build-up-and-fill structure: The depositional signature of strongly aggradational chute-and-pool bedforms Arnoud Slootman College of Petroleum Engineering & Geosciences, King Fahd University of Petroleum and Minerals, Dhahran, Saudi Arabia – [email protected] Matthieu J.B. Cartigny Department of Geography, Durham University, Durham, United Kingdom – [email protected] Age J. Vellinga National Oceanography Centre, University of Southampton Waterfront Campus, Southampton, United Kingdom – [email protected] ABSTRACT: Chute -and -pools are unstable, hybrid bedforms of the upper flo w-regime, pop u- lating the stability field in between two stable end-members (antidunes and cyclic steps). Chute-and-pools are manifested by supercritical flow (chute) down the lee side and subcritical flow (pool) on the stoss side, linked through a hydraulic jump in the trough. The associated sedimentary structures reported here were generated at the toe-of-slope of a Pleistocene car- bonate platform dominated by resedimentation of skeletal sand and gravel by supercritical density underflows. It is shown that wave-breaking on growing antidunes occurred without destruction of the antidune like commonly observed for antidunes in subaerial flows. This led to the formation of chute-and-pools that were not preceded by intense upstream scouring, in- terpreted to be the result of high bed aggradation rates. The term aggradational chute-and- pool is proposed for these -
Fluvial Sedimentary Patterns
ANRV400-FL42-03 ARI 13 November 2009 11:49 Fluvial Sedimentary Patterns G. Seminara Department of Civil, Environmental, and Architectural Engineering, University of Genova, 16145 Genova, Italy; email: [email protected] Annu. Rev. Fluid Mech. 2010. 42:43–66 Key Words First published online as a Review in Advance on sediment transport, morphodynamics, stability, meander, dunes, bars August 17, 2009 The Annual Review of Fluid Mechanics is online at Abstract fluid.annualreviews.org Geomorphology is concerned with the shaping of Earth’s surface. A major by University of California - Berkeley on 02/08/12. For personal use only. This article’s doi: contributing mechanism is the interaction of natural fluids with the erodible 10.1146/annurev-fluid-121108-145612 Annu. Rev. Fluid Mech. 2010.42:43-66. Downloaded from www.annualreviews.org surface of Earth, which is ultimately responsible for the variety of sedi- Copyright c 2010 by Annual Reviews. mentary patterns observed in rivers, estuaries, coasts, deserts, and the deep All rights reserved submarine environment. This review focuses on fluvial patterns, both free 0066-4189/10/0115-0043$20.00 and forced. Free patterns arise spontaneously from instabilities of the liquid- solid interface in the form of interfacial waves affecting either bed elevation or channel alignment: Their peculiar feature is that they express instabilities of the boundary itself rather than flow instabilities capable of destabilizing the boundary. Forced patterns arise from external hydrologic forcing affect- ing the boundary conditions of the system. After reviewing the formulation of the problem of morphodynamics, which turns out to have the nature of a free boundary problem, I discuss systematically the hierarchy of patterns observed in river basins at different scales. -
Role of Upper-Flow-Regime Bedforms Emplaced by Sediment Gravity Flows in the Evolution of Deltas
Journal of Marine Science and Engineering Review Role of Upper-Flow-Regime Bedforms Emplaced by Sediment Gravity Flows in the Evolution of Deltas Svetlana Kostic 1,*, Daniele Casalbore 2, Francesco Chiocci 2, Jörg Lang 3 and Jutta Winsemann 3 1 Computation Science Research Center, San Diego State University, San Diego, CA 92182, USA 2 Sapienza University of Rome, 00185 Rome, Italy; [email protected] (D.C.); [email protected] (F.C.) 3 Institute for Geology, Leibniz University Hannover, 30167 Hannover, Germany; [email protected] (J.L.); [email protected] (J.W.) * Correspondence: [email protected] Received: 20 November 2018; Accepted: 27 December 2018; Published: 4 January 2019 Abstract: Upper-flow-regime bedforms and their role in the evolution of marine and lacustrine deltas are not well understood. Wave-like undulations on delta foresets are by far the most commonly reported bedforms on deltas and it will take time before many of these features get identified as upper-flow-regime bedforms. This study aims at: (1) Providing a summary of our knowledge to date on deltaic bedforms emplaced by sediment gravity flows; (2) illustrating that these features are most likely transitional upper-flow-regime bedforms; and (3) using field case studies of two markedly different deltas in order to examine their role in the evolution of deltas. The study combines numerical analysis with digital elevation models, outcrop, borehole, and high-resolution seismic data. The Mazzarrà river delta in the Gulf of Patti, Italy, is selected to show that upper-flow-regime bedforms in gullies can be linked to the onset, growth, and evolution of marine deltas via processes of gully initiation, filling, and maintenance. -
Simons Et Al. 2004
Geomorphic, Hydrologic, Hydraulic and Sediment Concepts Applied To Alluvial Rivers By Daryl B. Simons, Ph.D., P.E., D.B. Simons & Associates, Inc.; Everett V. Richardson, Ph.D., P.E., Ayres Associates, Inc.; Maurice L. Albertson, Ph.D., P.E., Colorado State University; Robert J. Kodoatie, Ph.D., Diponegoro University, Indonesia. 2004 Daryl B. Simons Published by Colorado State University OPEN FILE INTERNET – FREE DOWNLOAD Dedicated to Major Contributors to the Concepts of Flow of Water and Sediment in Alluvial Channels: Paul C. Benedict, U.S. Geological Survey; Donald C. Bondurant, U.S. Corps of Engineers; Whitney M. Borland, U.S. Bureau of Reclamation; Bruce R. Colby, U.S. Geological Survey; Brynon C. Colby, U.S. Geological Survey; Hans A. Einstein, University of California, Berkeley; Dave W. Hubbell, U.S. Geological Survey; E.W. Lane, U.S. Bureau of Reclamation; Emmett M. Laursen, University of Arizona; Luna B. Leopold, U.S. Geological Survey; Carl F. Nordin, U.S. Geological Survey; Hunter Rouse, University of Iowa; Stanley E. Schumm, Colorado State University; Lorenzo G. Straub, University of Minnesota; and Vito A. Vanoni, California Institute of Technology. iii Table of Contents LIST OF SYMBOL ....................................................................................... V ABSTRACT ................................................................................................. IX 1. INTRODUCTION ................................................................................... 1 2. FUNDAMENTALS THAT MUST BE INTEGRATED INTO -
THE SAN JUAN CANYON H PUBLIC
DEPARTMENT OF THE INTERIOR HUBERT WORK, Secretary UNITED STATES GEOLOGICAL SURVEY GEORGE OTIS SMITH, Director Water-Supply Paper 538 THE SAN JUAN CANYON SOUTHEASTERN UTAH A GEOGRAPHIC AND HYDROGRAPHIC RECONNAISSANCE BY HUGH D. MISER h PUBLIC WASHINGTON GOVERNMENT PRINTING OFFICE 1924 ADDITIONAL COPIES OF THIS PUBLICATION MAT BE PROCURED FKOM THE SUPERINTENDENT OF DOCUMENTS GOVERNMENT PRINTING OFFICE WASHINGTON, D. C. AT 30 CENTS PER COPY CONTENTS Page Location-_________________________________ 1 Present and previous explorations________,________ 1 Acknowledgments ________________________________ 4 Suggestions to overland travelers____________________ 4 Geography _ _____ _ _______ _ _ _ 6 Surface features _________________________,____ & Bluff to Chinle Creek______________________ _ 7 Chinle Creek to Cedar Point__________________-_ 8 Cedar Point to Clay Hill Crossing__________________ 9 Clay Hill Crossing to Piute Farms__________________ 11 Piute Farms to mouth of river____________ _ _ __ 11 Climate____________________________________ 16 Precipitation and temperature___________________ 16 Wind_*._______________________________1_ 17 Soil_______________________________________ 18 Flora_______________________________________ 18 Animals __________________________________ 20 Mineral resources ______________________________ 21 Inhabitants_______________________________j._ 22 Irrigation and agriculture_________________________ 24 Archeology. ___________________________________ 25 Roads and trails ______________________________ 26 Geology_______________________________________ -
EGU2015-7279, 2015 EGU General Assembly 2015 © Author(S) 2015
Geophysical Research Abstracts Vol. 17, EGU2015-7279, 2015 EGU General Assembly 2015 © Author(s) 2015. CC Attribution 3.0 License. Supercritical strata in Lower Paleozoic fluvial rocks: a super critical link to upper flow regime processes and preservation in nature David Lowe and Bill Arnott Department of Earth Sciences, University of Ottawa, Ottawa, Ontario, Canada K1N 6N5 ([email protected]) Recent experimental work has much improved our understanding of the lithological attributes of open-channel supercritical flow deposits, namely those formed by antidunes, chutes-and-pools and cyclic steps. However their limited documentation in the ancient sedimentary record brings into question details about their geological preser- vation. Antidune, chute-and-pool and cyclic step deposits are well developed in sandy ephemeral fluvial deposits of the Upper Cambrian – Lower Ordovician Potsdam Group in the Ottawa Embayment of eastern North America. These high energy fluvial strata form dm- to a few m-thick units intercalated within thick, areally expansive succes- sions of sheet sandstones consisting mostly of wind ripple and adhesion stratification with common deflation lags. Collectively these strata record deposition in a semi-arid environment in which rare, episodic high-energy fluvial events accounted for most of the influx of sediment from upland sources. Following deposition, however, extensive aeolian processes reworked the sediment pile, and hence modified profoundly the preserved stratigraphic record. Antidune deposits occur as 0.2 – 1.6 m thick cosets made up of 2 – 15 cm thick lenticular sets of low angle (≤ 20o) cross-stratified, medium- to coarse-grained sandstone bounded by low-angle (5 – 15o) concave-upward scours and, in many cases, capped by low angle (10 – 15o) convex-upwards symmetrical formsets. -
Stream Dynamics Are Demonstrated Through Dis- Cussion of Processes and Process Indicators; Theory Is Included Only Where Helpful to Explain Concepts
This file was created by scanning the printed publication. Errors identified by the software have been corrected; however, some errors may remain. Abstract Concepts of stream dynamics are demonstrated through dis- cussion of processes and process indicators; theory is included only where helpful to explain concepts. Present knowledge allows only qualitative prediction of stream behavior. However, such pre- dictions show how management actions will affect the stream and its environment. USDA Forest Service April 1980 General Technical Report RM-72 Stream Dynamics: An Overview for Land Managers Burchard H. Heede, Principal Hydraulic Engineer Rocky Mountain Forest and Range Experiment Station1 'Headquarters at Fort Collins, in cooperation with Colorado State University. Research reported here was conducted at the Station's Research Work Unit at Tempe, in cooperation with Arizona State University. Contents Introduction ..................................................................... Scope and Purpose ............................................................. Characteristics of Streams ...................................................... The Basic Fluvial Process ......................................................... Subcritical Versus Supercritical Flow ............................................ Froude Number .............................................................. Laminar Versus Turbulent Flow ..........................................;....... Reynolds Number ........................................................... -
Morphology and Morphodynamics of Braided Rivers: an Experimental Investigation
Thèse n° 7504 Morphology and morphodynamics of braided rivers: an experimental investigation Présentée le 13 octobre 2020 à la Faculté de l’environnement naturel, architectural et construit Laboratoire d’hydraulique environnementale Programme doctoral en mécanique pour l’obtention du grade de Docteur ès Sciences par Daniel Vito PAPA Acceptée sur proposition du jury Prof. F. Gallaire, président du jury Prof. C. Ancey, directeur de thèse Prof. M. G. Kleinhans, rapporteur Dr P. Bohórquez Rodríguez de Medina, rapporteur Dr G. De Cesare, rapporteur 2020 Be realistic, demand the impossible! — Herbert Marcuse Alea jiacta est — Caius Iulius Caesar Remerciements Par où commencer la partie, probablement la plus dicile, d’une thèse ? Qui remercier en premier ? En dernier ? Ai-je oublié quelqu’un ? Va-t-il/elle se fâcher si je ne le/la mentionne pas ? Est-ce trop long ? Est-ce trop court ? Est-ce bien écrit ? Bon, pour ne pas rater ce dernier grand moment de science, je vais commencer par le début, à savoir par El Jefe. Merci Christophe de m’avoir donné l’opportunité de réaliser cette thèse. Je ne sais pas trop où tu avais la tête en m’engageant, mais au nal ça s’est plutôt bien terminé. On aura au moins pu discuter de pleins de sujets qui n’avaient pas grand-chose à voir avec ma thèse (et c’est tant mieux d’ailleurs), tu m’auras fait découvrir pleins de livres et d’auteurs que je n’aurais jamais connu sans toi. Et moi, ben on va dire que j’ai rafraîchi ton espagnol. -
An Outcrop Study of Bedding Features at Outcrop Scale
DETERMINING THE DEPOSITIONAL ENVIRONMENT OF THE LOWER EAGLE FORD IN LOZIER CANYON, ANTONIO CREEK, AND OSMAN CANYON, TEXAS: AN OUTCROP STUDY OF BEDDING FEATURES AT OUTCROP SCALE A Thesis by TREY SAXON LYON Submitted to the Office of Graduate and Professional Studies of Texas A&M University in partial fulfillment of the requirements for the degree of MASTER OF SCIENCE Chair of Committee, John R. Giardino Committee Members, Michael C. Pope Juan Carlos Laya Chris Houser Head of Department, John R. Giardino May 2015 Major Subject: Geology Copyright 2015 Trey Saxon Lyon ABSTRACT The Eagle Ford Formation is currently the most economically significant unconventional resource play in the state of Texas. There has been much debate as to the environment of deposition for the lowermost Facies A of the Eagle Ford in outcrop exposures in Lozier Canyon, Texas. Two conflicting hypotheses were proposed: 1) Sedimentary structures in Facies A are hummocky cross-stratification (HCS) and swaley cross-stratification (SCS), which indicates a shelfal depositional environment above the storm wave base (SWB). 2) Sedimentary structures in Facies A are a mixture of diagenetically separated contourites, turbidites, and pinch-and-swell beds, which indicate a distal-slope depositional environment below SWB. This research used field work, three-dimensional analysis of sedimentary structures, measurements of ripple height, and laboratory analysis to interpret the environment of deposition. The results of these observations and data suggest that the sedimentary structures in Facies A record a depositional environment above SWB. Observation of cross-bedded structures in three-dimensions reveals (i) isotropic truncation of laminae; (ii) symmetric rounded ripples; (iii) large variations in laminae geometry, truncation, and dip inclination, attributed to fluctuations in storm intensity, frequency, and duration; and (iv) and bidirectional downlap and reactivation surfaces associated with oscillatory flow above the SWB. -
Guidance for Stream Restoration
United States Department of Agriculture Guidance for Stream Restoration Steven E. Yochum Forest National Stream & Technical Note Service Aquatic Ecology Center TN-102.4 May 2018 Yochum, Steven E. 2018. Guidance for Stream Restoration. U.S. Department of Agriculture, Forest Service, National Stream & Aquatic Ecology Center, Technical Note TN-102.4. Fort Collins, CO. Cover Photos: Top-right: Illinois River, North Park, Colorado. Photo by Steven Yochum Bottom-left: Whychus Creek, Oregon. Photo by Paul Powers ABSTRACT A great deal of effort has been devoted to developing guidance for stream restoration. The available resources are diverse, reflecting the wide ranging approaches used and expertise required to develop effective stream restoration projects. To help practitioners sort through the extensive information, this technical note has been developed to provide a guide to the available guidance. The document structure is primarily a series of short literature reviews followed by a hyperlinked reference list for readers to find more information on each topic. The primary topics incorporated into this guidance include general methods, an overview of stream processes and restoration, case studies, data compilation, preliminary assessments, and field data collection. Analysis methods and tools, and planning and design guidance for specific restoration features are also provided. This technical note is a bibliographic repository of information available to assist professionals with the process of planning, analyzing, and designing stream restoration projects. U.S. Forest Service NSAEC TN-102.4 Fort Collins, Colorado Guidance for Stream Restoration & Rehabilitation i of vi May 2018 ADVISORY NOTE Techniques and approaches contained in this technical note are not all-inclusive, nor universally applicable. -
Step–Pool Evolution in the Rio Cordon, Northeastern Italy
Earth Surface Processes and Landforms Earth Surf. Process. Landforms 26, 991–1008 (2001) DOI: 10.1002/esp.239 STEP–POOL EVOLUTION IN THE RIO CORDON, NORTHEASTERN ITALY MARIO A. LENZI* Department of Land and Agro-Forest Environments, University of Padova, Agripolis, via Romea, 35020 Legnaro (PD), Italy Received 4 September 2000; Revised 20 February 2001; Accepted 9 March 2001 ABSTRACT The principle that formative events, punctuated by periods of evolution, recovery or temporary periods of steady-state conditions, control the development of the step–pool morphology, has been applied to the evolution of the Rio Cordon stream bed. The Rio Cordon is a small catchment (5 km2) within the Dolomites wherein hydraulic parameters of floods and the coarse bedload are recorded. Detailed field surveys of the step–pool structures carried out before and after the September 1994 and October 1998 floods have served to illustrate the control on step–pool changes by these floods. Floods were grouped into two categories. The first includes ‘ordinary’ events which are characterized by peak discharges with a return time of one to five years (1Ð8–5Ð15 m3 s1) and by an hourly bedload rate not exceeding 20 m3 h1. The second refers to ‘exceptional’ events with a return time of 30–50 years. A flood of this latter type occurred on 14 September 1994, with a peak discharge of 10Ð4m3 s1 and average hourly bedload rate of 324 m3 h1. Step–pool features were characterized primarily by a steepness parameter c D H/Ls/S. The evolution of the steepness parameter was measured in the field from 1992 to 1998. -
Morphodynamics and Sedimentary Structures Of
1 1 Running head: Dynamics and structures of supercritical-flow bedforms 2 3 Morphodynamics and sedimentary structures of 4 bedforms under supercritical-flow conditions: new 5 insights from flume experiments 6 7 MATTHIEU J.B. CARTIGNY*, DARIO VENTRA, GEORGE POSTMA 8 and JAN H. VAN DEN BERG 9 10 Faculty of Geosciences, Utrecht University, P.O. box 80021, 11 3508TA Utrecht, The Netherlands 12 * Corresponding author current address: National Oceanography Centre, European Way, 13 Southampton, Hampshire, UK SO14 3ZH (E-mail: [email protected]) 14 15 16 17 18 19 20 21 22 23 Keywords Supercritical flow, cyclic steps, antidunes, hydraulic jump, chutes-and-pools, 24 flume experiments 25 26 2 27 ABSTRACT 28 Supercritical flow phenomena are fairly common in modern sedimentary environments, yet their 29 recognition and analysis remain difficult in the stratigraphic record. This fact is commonly 30 ascribed to the poor preservation potential of deposits from high-energy supercritical flows. 31 However, the number of flume datasets on supercritical flow dynamics and sedimentary 32 structures is very limited in comparison with available data for subcritical flows, which hampers 33 the recognition and interpretation of such deposits. The results of systematic flume experiments 34 spanning the full range of supercritical flow bedforms (antidunes, chutes-and-pools, cyclic steps) 35 developed in mobile sand beds of variable grain sizes are presented. Flow character and 36 related bedform patterns are constrained through time-series measurements of bed 37 configurations, flow depths, flow velocities and Froude numbers. The results allow the 38 refinement and extension of some widely used bedform stability diagrams in the supercritical- 39 flow domain, clarifying in particular the morphodynamic relations between antidunes and cyclic 40 steps.