And Mn-Dependent Dioxygenases: Evidence for Oxygen Activation Without a Change in Metal Redox State
Total Page:16
File Type:pdf, Size:1020Kb
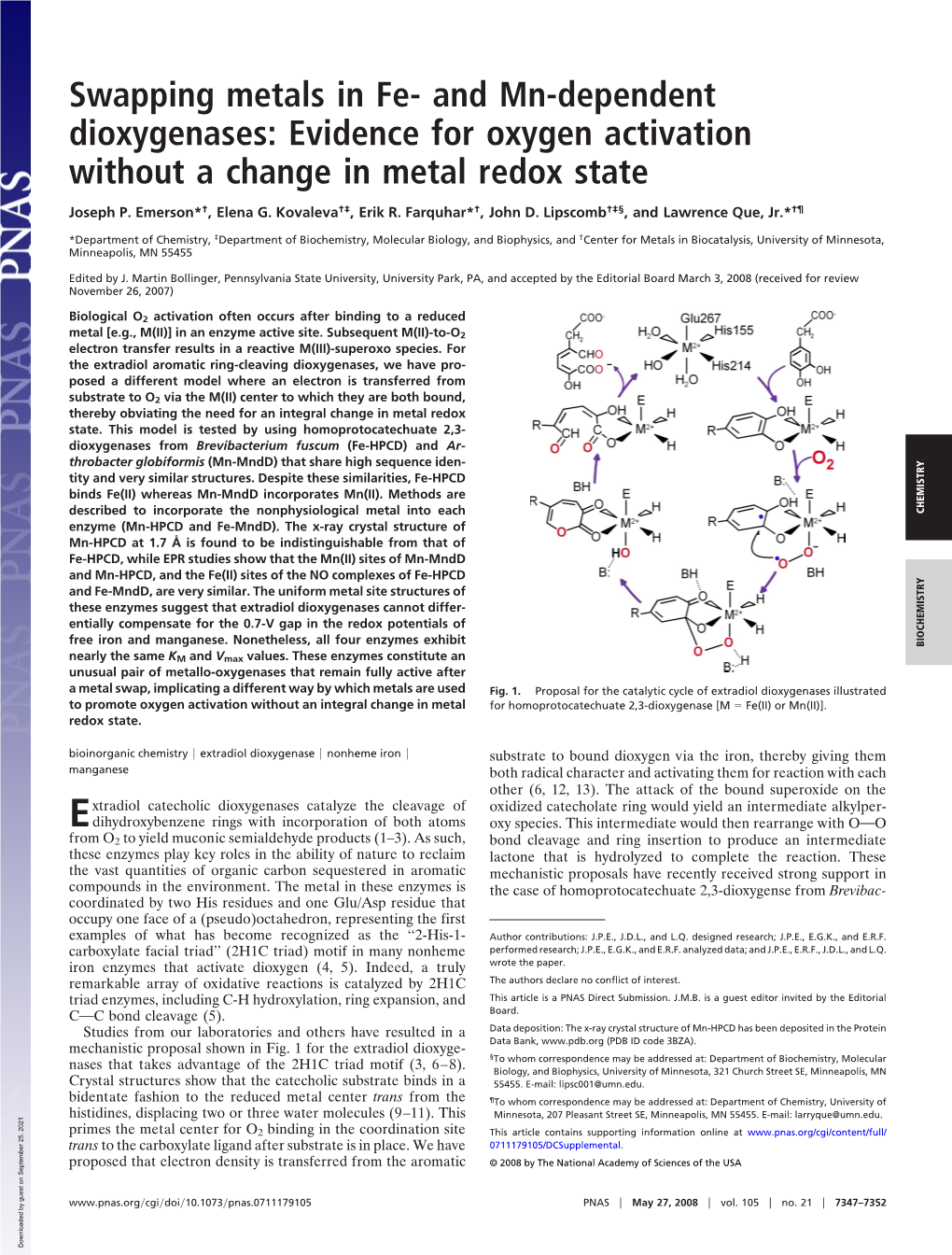
Load more
Recommended publications
-
Bioinorganic Chemistry of Nickel
inorganics Editorial Bioinorganic Chemistry of Nickel Michael J. Maroney 1,* and Stefano Ciurli 2,* 1 Department of Chemistry and Program in Molecular and Cellular Biology, University of Massachusetts Amherst, 240 Thatcher Rd. Life Sciences, Laboratory Rm N373, Amherst, MA 01003, USA 2 Laboratory of Bioinorganic Chemistry, Department of Pharmacy and Biotechnology, University of Bologna, Viale G. Fanin 40, I-40127 Bologna, Italy * Correspondence: [email protected] (M.J.M.); [email protected] (S.C.) Received: 11 October 2019; Accepted: 11 October 2019; Published: 30 October 2019 Following the discovery of the first specific and essential role of nickel in biology in 1975 (the dinuclear active site of the enzyme urease) [1], nickel has become a major player in bioinorganic chemistry,particularly in microorganisms, having impacts on both environmental settings and human pathologies. At least nine classes of enzymes are now known to require nickel in their active sites, including catalysis of redox [(Ni,Fe) hydrogenases, carbon monoxide dehydrogenase, methyl coenzyme M reductase, acetyl coenzyme A synthase, superoxide dismutase] and nonredox (glyoxalase I, acireductone dioxygenase, lactate isomerase, urease) chemistries. In addition, the dark side of nickel has been illuminated in regard to its participation in microbial pathogenesis, cancer, and immune responses. Knowledge gleaned from the investigations of inorganic chemists into the coordination and redox chemistry of this element have boosted the understanding of these biological roles of nickel in each context. In this issue, eleven contributions, including four original research articles and seven critical reviews, will update the reader on the broad spectrum of the role of nickel in biology. -
Cysteine Dioxygenase 1 Is a Metabolic Liability for Non-Small Cell Lung Cancer Authors: Yun Pyo Kang1, Laura Torrente1, Min Liu2, John M
bioRxiv preprint doi: https://doi.org/10.1101/459602; this version posted November 1, 2018. The copyright holder for this preprint (which was not certified by peer review) is the author/funder. All rights reserved. No reuse allowed without permission. Cysteine dioxygenase 1 is a metabolic liability for non-small cell lung cancer Authors: Yun Pyo Kang1, Laura Torrente1, Min Liu2, John M. Asara3,4, Christian C. Dibble5,6 and Gina M. DeNicola1,* Affiliations: 1 Department of Cancer Physiology, H. Lee Moffitt Cancer Center and Research Institute, Tampa, FL, USA 2 Proteomics and Metabolomics Core Facility, Moffitt Cancer Center and Research Institute, Tampa, FL, USA 3 Division of Signal Transduction, Beth Israel Deaconess Medical Center, Boston, MA, USA 4 Department of Medicine, Harvard Medical School, Boston, MA, USA 5 Department of Pathology and Cancer Center, Beth Israel Deaconess Medical Center, Boston, MA, USA 6 Department of Pathology, Harvard Medical School, Boston, MA, USA *Correspondence to: [email protected]. Keywords: KEAP1, NRF2, cysteine, CDO1, sulfite Summary NRF2 is emerging as a major regulator of cellular metabolism. However, most studies have been performed in cancer cells, where co-occurring mutations and tumor selective pressures complicate the influence of NRF2 on metabolism. Here we use genetically engineered, non-transformed primary cells to isolate the most immediate effects of NRF2 on cellular metabolism. We find that NRF2 promotes the accumulation of intracellular cysteine and engages the cysteine homeostatic control mechanism mediated by cysteine dioxygenase 1 (CDO1), which catalyzes the irreversible metabolism of cysteine to cysteine sulfinic acid (CSA). Notably, CDO1 is preferentially silenced by promoter methylation in non-small cell lung cancers (NSCLC) harboring mutations in KEAP1, the negative regulator of NRF2. -
Indoleamine 2,3-Dioxygenase and Its Therapeutic Inhibition in Cancer George C
View metadata, citation and similar papers at core.ac.uk brought to you by CORE provided by Scholarship, Research, and Creative Work at Bryn Mawr College | Bryn Mawr College... Bryn Mawr College Scholarship, Research, and Creative Work at Bryn Mawr College Chemistry Faculty Research and Scholarship Chemistry 2018 Indoleamine 2,3-Dioxygenase and Its Therapeutic Inhibition in Cancer George C. Prendergast William Paul Malachowski Bryn Mawr College, [email protected] Arpita Mondal Peggy Scherle Alexander J. Muller Let us know how access to this document benefits ouy . Follow this and additional works at: https://repository.brynmawr.edu/chem_pubs Part of the Chemistry Commons Custom Citation George C. Prendergast, William J. Malachowski, Arpita Mondal, Peggy Scherle, and Alexander J. Muller. 2018. "Indoleamine 2,3-Dioxygenase and Its Therapeutic Inhibition in Cancer." International Review of Cell and Molecular Biology 336: 175-203. This paper is posted at Scholarship, Research, and Creative Work at Bryn Mawr College. https://repository.brynmawr.edu/chem_pubs/25 For more information, please contact [email protected]. Indoleamine 2,3-Dioxygenase and Its Therapeutic Inhibition in Cancer George C. Prendergast, William, Malachowski, Arpita Mondal, Peggy Scherle, and Alexander J. Muller International Review of Cell and Molecular Biology 336: 175-203. http://doi.org/10.1016/bs.ircmb.2017.07.004 ABSTRACT The tryptophan catabolic enzyme indoleamine 2,3-dioxygenase-1 (IDO1) has attracted enormous attention in driving cancer immunosuppression, neovascularization, and metastasis. IDO1 suppresses local CD8+ T effector cells and natural killer cells and induces CD4+ T regulatory cells (iTreg) and myeloid-derived suppressor cells (MDSC). The structurally distinct enzyme tryptophan dioxygenase (TDO) also has been implicated recently in immune escape and metastatic progression. -
Muscle Regeneration Controlled by a Designated DNA Dioxygenase
Wang et al. Cell Death and Disease (2021) 12:535 https://doi.org/10.1038/s41419-021-03817-2 Cell Death & Disease ARTICLE Open Access Muscle regeneration controlled by a designated DNA dioxygenase Hongye Wang1, Yile Huang2,MingYu3,YangYu1, Sheng Li4, Huating Wang2,5,HaoSun2,5,BingLi 3, Guoliang Xu6,7 andPingHu4,8,9 Abstract Tet dioxygenases are responsible for the active DNA demethylation. The functions of Tet proteins in muscle regeneration have not been well characterized. Here we find that Tet2, but not Tet1 and Tet3, is specifically required for muscle regeneration in vivo. Loss of Tet2 leads to severe muscle regeneration defects. Further analysis indicates that Tet2 regulates myoblast differentiation and fusion. Tet2 activates transcription of the key differentiation modulator Myogenin (MyoG) by actively demethylating its enhancer region. Re-expressing of MyoG in Tet2 KO myoblasts rescues the differentiation and fusion defects. Further mechanistic analysis reveals that Tet2 enhances MyoD binding by demethylating the flanking CpG sites of E boxes to facilitate the recruitment of active histone modifications and increase chromatin accessibility and activate its transcription. These findings shed new lights on DNA methylation and pioneer transcription factor activity regulation. Introduction Ten-Eleven Translocation (Tet) family of DNA dioxy- 1234567890():,; 1234567890():,; 1234567890():,; 1234567890():,; Skeletal muscles can regenerate due to the existence of genases catalyze the active DNA demethylation and play muscle stem cells (MuSCs)1,2. The normally quiescent critical roles in embryonic development, neural regen- MuSCs are activated after muscle injury and further dif- eration, oncogenesis, aging, and many other important – ferentiate to support muscle regeneration3,4. -
Relating Metatranscriptomic Profiles to the Micropollutant
1 Relating Metatranscriptomic Profiles to the 2 Micropollutant Biotransformation Potential of 3 Complex Microbial Communities 4 5 Supporting Information 6 7 Stefan Achermann,1,2 Cresten B. Mansfeldt,1 Marcel Müller,1,3 David R. Johnson,1 Kathrin 8 Fenner*,1,2,4 9 1Eawag, Swiss Federal Institute of Aquatic Science and Technology, 8600 Dübendorf, 10 Switzerland. 2Institute of Biogeochemistry and Pollutant Dynamics, ETH Zürich, 8092 11 Zürich, Switzerland. 3Institute of Atmospheric and Climate Science, ETH Zürich, 8092 12 Zürich, Switzerland. 4Department of Chemistry, University of Zürich, 8057 Zürich, 13 Switzerland. 14 *Corresponding author (email: [email protected] ) 15 S.A and C.B.M contributed equally to this work. 16 17 18 19 20 21 This supporting information (SI) is organized in 4 sections (S1-S4) with a total of 10 pages and 22 comprises 7 figures (Figure S1-S7) and 4 tables (Table S1-S4). 23 24 25 S1 26 S1 Data normalization 27 28 29 30 Figure S1. Relative fractions of gene transcripts originating from eukaryotes and bacteria. 31 32 33 Table S1. Relative standard deviation (RSD) for commonly used reference genes across all 34 samples (n=12). EC number mean fraction bacteria (%) RSD (%) RSD bacteria (%) RSD eukaryotes (%) 2.7.7.6 (RNAP) 80 16 6 nda 5.99.1.2 (DNA topoisomerase) 90 11 9 nda 5.99.1.3 (DNA gyrase) 92 16 10 nda 1.2.1.12 (GAPDH) 37 39 6 32 35 and indicates not determined. 36 37 38 39 S2 40 S2 Nitrile hydration 41 42 43 44 Figure S2: Pearson correlation coefficients r for rate constants of bromoxynil and acetamiprid with 45 gene transcripts of ECs describing nucleophilic reactions of water with nitriles. -
ALOX12 Antibody
Efficient Professional Protein and Antibody Platforms ALOX12 Antibody Basic information: Catalog No.: UPA60470 Source: Rabbit Size: 50ul/100ul Clonality: polyclonal Concentration: 1mg/ml Isotype: Rabbit IgG Purification: affinity purified by Protein A Useful Information: Applications: WB:1:500-2000 Reactivity: Human, Mouse, Rat, Cow Specificity: This antibody recognizes ALOX12 protein. KLH conjugated synthetic peptide derived from human 12 Lipoxygenase Immunogen: 101-200/663 Non-heme iron-containing dioxygenase that catalyzes the stereo-specific peroxidation of free and esterified polyunsaturated fatty acids generating a spectrum of bioactive lipid mediators. Mainly converts arachidonic acid to (12S)-hydroperoxyeicosatetraenoic acid/(12S)-HPETE but can also metabo- lize linoleic acid. Has a dual activity since it also converts leukotriene A4/LTA4 into both the bioactive lipoxin A4/LXA4 and lipoxin B4/LXB4. Description: Through the production of specific bioactive lipids like (12S)-HPETE it regu- lates different biological processes including platelet activation. It also probably positively regulates angiogenesis through regulation of the expres- sion of the vascular endothelial growth factor. Plays a role in apoptotic pro- cess, promoting the survival of vascular smooth muscle cells for instance. May also play a role in the control of cell migration and proliferation. {ECO:0000269|PubMed:16638750, Uniprot: P18054 Human BiowMW: 76 KDa Buffer: 0.01M TBS(pH7.4) with 1% BSA, 0.03% Proclin300 and 50% Glycerol. Storage: Store at 4°C short term and -20°C long term. Avoid freeze-thaw cycles. Note: For research use only, not for use in diagnostic procedure. Data: Gene Universal Technology Co. Ltd www.universalbiol.com Tel: 0550-3121009 E-mail: [email protected] Efficient Professional Protein and Antibody Platforms Sample: Raw264.7 Cell Lysate at 40 ug A431 Cell Lysate at 40 ug Primary: Anti-ALOX12 at 1/300 dilution Secondary: IRDye800CW Goat An- ti-Rabbit IgG at 1/20000 dilution Predicted band size: 73 kD Observed band size: 73 kD Gene Universal Technology Co. -
Mechanistic Study of Cysteine Dioxygenase, a Non-Heme
MECHANISTIC STUDY OF CYSTEINE DIOXYGENASE, A NON-HEME MONONUCLEAR IRON ENZYME by WEI LI Presented to the Faculty of the Graduate School of The University of Texas at Arlington in Partial Fulfillment of the Requirements for the Degree of DOCTOR OF PHILOSOPHY THE UNIVERSITY OF TEXAS AT ARLINGTON August 2014 Copyright © by Student Name Wei Li All Rights Reserved Acknowledgements I would like to thank Dr. Pierce for your mentoring, guidance and patience over the five years. I cannot go all the way through this without your help. Your intelligence and determination has been and will always be an example for me. I would like to thank my committee members Dr. Dias, Dr. Heo and Dr. Jonhson- Winters for the directions and invaluable advice. I also would like to thank all my lab mates, Josh, Bishnu ,Andra, Priyanka, Eleanor, you all helped me so I could finish my projects. I would like to thank the Department of Chemistry and Biochemistry for the help with my academic and career. At Last, I would like to thank my lovely wife and beautiful daughter who made my life meaningful and full of joy. July 11, 2014 iii Abstract MECHANISTIC STUDY OF CYSTEINE DIOXYGENASE A NON-HEME MONONUCLEAR IRON ENZYME Wei Li, PhD The University of Texas at Arlington, 2014 Supervising Professor: Brad Pierce Cysteine dioxygenase (CDO) is an non-heme mononuclear iron enzymes that catalyzes the O2-dependent oxidation of L-cysteine (Cys) to produce cysteine sulfinic acid (CSA). CDO controls cysteine levels in cells and is a potential drug target for some diseases such as Parkinson’s and Alzhermer’s. -
Catalytic Strategies of the Non-Heme Iron Dependent Oxygenases And
Available online at www.sciencedirect.com ScienceDirect Catalytic strategies of the non-heme iron dependent oxygenases and their roles in plant biology Mark D White and Emily Flashman Non-heme iron-dependent oxygenases catalyse the these enzymes play in plant biology. Targeted manipula- incorporation of O2 into a wide range of biological molecules tion of these enzymes could have beneficial effects for and use diverse strategies to activate their substrates. Recent plant growth and/or stress tolerance, addressing the 21st kinetic studies, including in crystallo, have provided century issue of food security. We therefore highlight experimental support for some of the intermediates used by those of potential agricultural (and/or health) interest. different subclasses of this enzyme family. Plant non-heme iron-dependent oxygenases have diverse and important Resolving intermediates in non-heme iron biological roles, including in growth signalling, stress dependent oxygenase catalysis responses and secondary metabolism. Recently identified To activate O2, non-heme iron-dependent oxygenases use roles include in strigolactone biosynthesis, O-demethylation in a redox active iron, coordinated octahedrally at their active morphine biosynthesis and regulating the stability of hypoxia- site with water, usually in a vicinal facial triad arrangement responsive transcription factors. We discuss current structural [1]. During turnover, H2O is sequentially displaced by and mechanistic understanding of plant non-heme iron substrate/co-substrate and O2, which is reductively acti- oxygenases, and how their chemical/genetic manipulation vated enabling a specific substrate modification. Enzyme could have agricultural benefit, for example, for improved yield, subfamilies are proposed to employ different reactive stress tolerance or herbicide development. -
Unique Coupling of Mono- and Dioxygenase Chemistries in a Single Active Site Promotes Heme Degradation
Unique coupling of mono- and dioxygenase chemistries in a single active site promotes heme degradation Toshitaka Matsuia,1, Shusuke Nambua, Celia W. Gouldingb,c, Satoshi Takahashia, Hiroshi Fujiid, and Masao Ikeda-Saitoa,1 aInstitute of Multidisciplinary Research for Advanced Materials, Tohoku University, Katahira, Aoba, Sendai 980-8577, Japan; bDepartment of Molecular Biology and Biochemistry, University of California, Irvine, CA 92697; cDepartment of Pharmaceutical Sciences, University of California, Irvine, CA 92697; and dDepartment of Chemistry, Graduate School of Humanities and Sciences, Nara Women’s University, Kitauoyanishi, Nara 630-8506, Japan Edited by Harry B. Gray, California Institute of Technology, Pasadena, CA, and approved February 26, 2016 (received for review November 26, 2015) − + Bacterial pathogens must acquire host iron for survival and colo- RH + O2 + 2e + 2H → ROH + H2O, [1] nization. Because free iron is restricted in the host, numerous pathogens have evolved to overcome this limitation by using a R + O → RðOÞ . [2] family of monooxygenases that mediate the oxidative cleavage of 2 2 heme into biliverdin, carbon monoxide, and iron. However, the Biological heme degradation proceeds through a unique self- etiological agent of tuberculosis, Mycobacterium tuberculosis, ac- oxidation mechanism where the substrate heme activates O complishes this task without generating carbon monoxide, which 2 potentially induces its latent state. Here we show that this unusual molecules. The canonical enzyme, heme oxygenase (HO), de- heme degradation reaction proceeds through sequential mono- grades heme into ferrous iron, carbon monoxide (CO), and bil- and dioxygenation events within the single active center of MhuD, iverdin by three successive monooxygenation reactions (Fig. 1A) a mechanism unparalleled in enzyme catalysis. -
Original Article Genetic Variants of Aloxs Genes in Polyunsaturated Fatty Acid/Arachidonic Acid Metabolism Associated with Type-2 Diabetes Development
Int J Clin Exp Med 2018;11(12):13797-13805 www.ijcem.com /ISSN:1940-5901/IJCEM0077987 Original Article Genetic variants of ALOXs genes in polyunsaturated fatty acid/arachidonic acid metabolism associated with type-2 diabetes development Jim Jinn-Chyuan Sheu1,3,4,5*, Ying-Ju Lin1,3*, Cherry Yin-Yi Chang2, Shih-Yin Chen1,3, Wen-Ling Liao1,4, Jai-Sing Yang1, Ming-Tsung Lai6, Chih-Mei Chen1, Chun-Cheng Tseng4, Tritium Hwang4, Ping-Ho Chen7, Fuu-Jen Tsai1,3 1Human Genetic Center, 2Department of Obstetrics and Gynecology, China Medical University Hospital, Taichung, Taiwan; 3School of Chinese Medicine, China Medical University, Taichung, Taiwan; 4Institute of Biomedical Sci- ences, National Sun Yat-sen University, Kaohsiung, Taiwan; 5Department of Health and Nutrition Biotechnology, Asia University, Taichung, Taiwan; 6Department of Pathology, Taichung Hospital, Ministry of Health and Welfare, Taichung, Taiwan; 7School of Dentistry, Kaohsiung Medical University, Kaohsiung, Taiwan. *Equal contributors. Received April 16, 2018; Accepted July 24, 2018; Epub December 15, 2018; Published December 30, 2018 Abstract: Poly-unsaturated fatty acids (PUFAs)/arachidonic acids (AAs) and their derived eicosanoids play potent roles in triggering inflammation during obesity and diabetes development. Recent studies have indicated functional roles of ALOX5, ALOX12, ALOX12B, and ALOX15 in the development of insulin resistance and islet β-cell dysfunc- tion. However, the impact of their genetic variants on type 2 diabetes (T2D) development in Asian patients remains unclear. In this study, 1,682 healthy controls and 788 patients with T2D were enrolled for genotyping those four ALOX genes by the TaqMan method. A total of eight Han Chinese-specific SNPs (two SNps for each gene) were selected for this study. -
Catalytic Triad' in Directing Promiscuous Substrate-Specificity
Spectroscopic and mechanistic investigation of the enzyme mercaptopropionic acid dioxygenase (MDO), the role of the conserved outer-sphere 'catalytic triad' in directing promiscuous substrate-specificity A DISSERTATION Presented to the faculty of the Graduate School of The University of Texas at Arlington in Partial fulfillment Of the requirements of the Degree of Doctor in Philosophy in CHEMISTRY by SINJINEE SARDAR Under the guidance of Dr. Brad S. Pierce 2nd May, 2018 ACKNOWLEDGEMENT It gives me immense pleasure to thank my compassionate guide Dr. Brad S. Pierce, for his exemplary guidance to accomplish this work. It has been an extraordinary pleasure and privilege to work under his guidance for the past few years. I express my sincere gratitude to my doctoral committee members Prof. Frederick. MacDonnell, Dr. Rasika Dias, and Dr. Jongyun Heo for their valuable advice and constant help. I would also like to thank the Department of Chemistry and Biochemistry of UTA for bestowing me the opportunity to have access to all the departmental facilities required for my work. Here, I express my heartiest gratitude to the ardent and humane guidance of my senior lab mates Dr. Josh Crowell and Dr. Bishnu Subedi which was indispensable. I am immeasurably thankful to my lab mates Wei, Mike, Phil, Nick, Jared and Sydney for their constant support and help. It has been a pleasure to working with all of you. The jokes and laughter we shared have reduced the hardships and stress of graduate school and had made the stressful journey fun. To end with, I utter my heartfelt gratefulness to my parents and my sister for their lifelong support and encouragement which helped me chase my dreams and aspirations and sincere appreciation towards my friends who are my extended family for their lively and spirited encouragement. -
Structural Enzymology of Sulfide Oxidation by Persulfide Dioxygenase and Rhodanese
Structural Enzymology of Sulfide Oxidation by Persulfide Dioxygenase and Rhodanese by Nicole A. Motl A dissertation submitted in partial fulfillment of the requirements for the degree of Doctor of Philosophy (Biological Chemistry) in the University of Michigan 2017 Doctoral Committee Professor Ruma Banerjee, Chair Assistant Professor Uhn-Soo Cho Professor Nicolai Lehnert Professor Stephen W. Ragsdale Professor Janet L. Smith Nicole A. Motl [email protected] ORCID iD: 0000-0001-6009-2988 © Nicole A. Motl 2017 ACKNOWLEDGEMENTS I would like to take this opportunity to acknowledge the many people who have provided me with guidance and support during my doctoral studies. First I would like to express my appreciation and gratitude to my advisor Dr. Ruma Banerjee for the mentorship, guidance, support and encouragement she has provided. I would like to thank my committee members Dr. Uhn-Soo Cho, Dr. Nicolai Lehnert, Dr. Stephen Ragsdale and Dr. Janet Smith for their advice, assistance and support. I would like to thank Dr. Janet Smith and members of Dr. Smith’s lab, especially Meredith Skiba, for sharing their expertise in crystallography. I would like to thank Dr. Omer Kabil for his help, suggestions and discussions in various aspects of my study. I would also like to thank members of Dr. Banerjee’s lab for their suggestions and discussions. Additionally, I would like to thank my friends and family for their support. ii TABLE OF CONTENTS ACKNOWLEDGEMENTS ii LIST OF TABLES viii LIST OF FIGURES ix ABBREVIATIONS xi ABSTRACT xii CHAPTER I. Introduction: