Adaptive Reservoir Operation Strategies Under Changing Boundary Conditions the Case of Aswan High Dam Reservoir
Total Page:16
File Type:pdf, Size:1020Kb
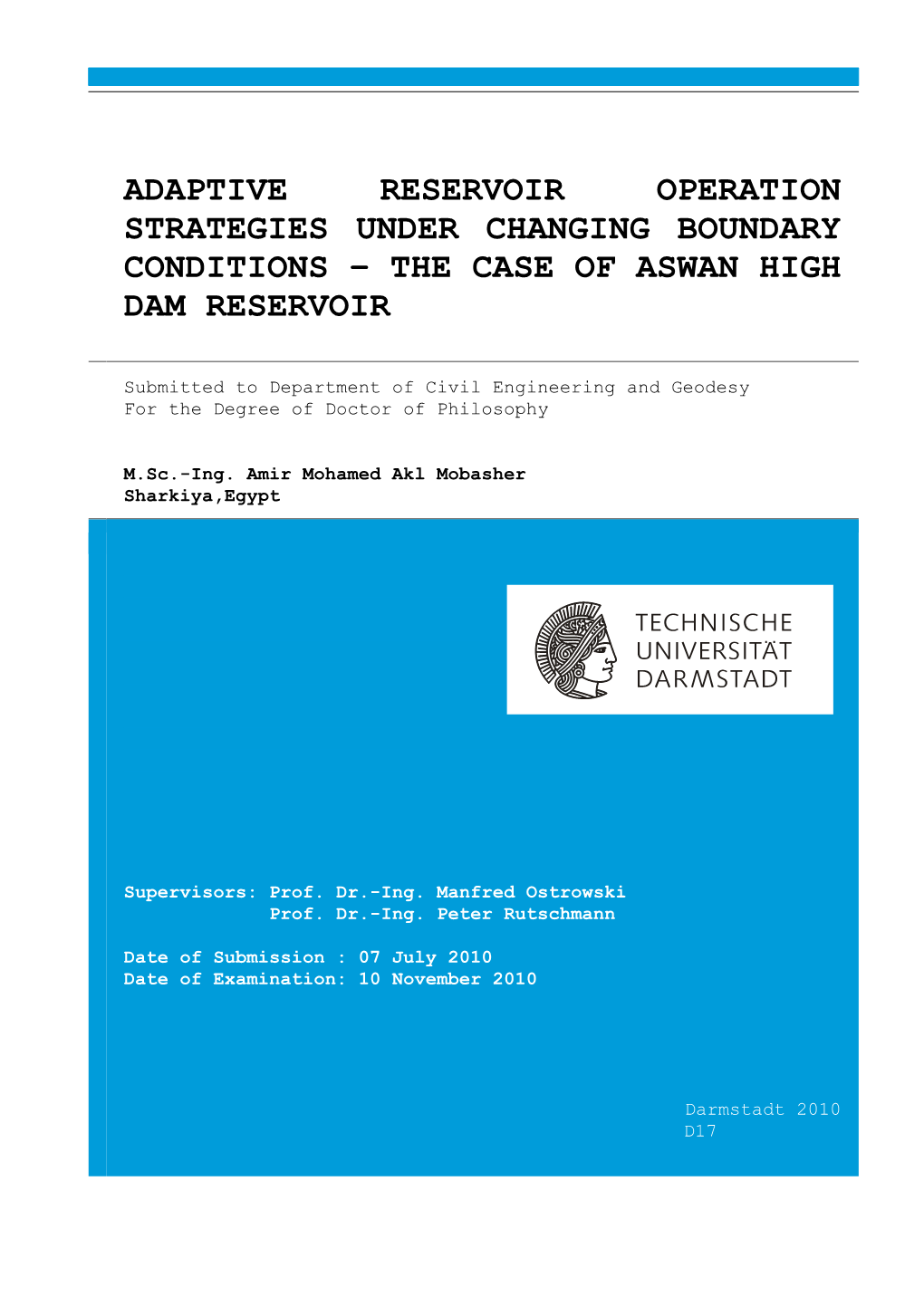
Load more
Recommended publications
-
A Governor of Dakhleh Oasis in the Early Middle Kingdom
EGYPTIAN CULTUR E AND SO C I E TY EGYPTIAN CULTUR E AND SO C I E TY S TUDI es IN HONOUR OF NAGUIB KANAWATI SUPPLÉMENT AUX ANNALES DU SERVICE DES ANTIQUITÉS DE L'ÉGYPTE CAHIER NO 38 VOLUM E I Preface by ZAHI HAWA ss Edited by AL E XANDRA WOOD S ANN MCFARLAN E SU S ANN E BIND E R PUBLICATIONS DU CONSEIL SUPRÊME DES ANTIQUITÉS DE L'ÉGYPTE Graphic Designer: Anna-Latifa Mourad. Director of Printing: Amal Safwat. Front Cover: Tomb of Remni. Opposite: Saqqara season, 2005. Photos: Effy Alexakis. (CASAE 38) 2010 © Conseil Suprême des Antiquités de l'Égypte All rights reserved. No part of this publication may be reproduced, stored in a retrieval system, or transmitted in any form or by any means, electronic, mechanical, photocopying, recording or other- wise, without the prior written permission of the publisher Dar al Kuttub Registration No. 2874/2010 ISBN: 978-977-479-845-6 IMPRIMERIE DU CONSEIL SUPRÊME DES ANTIQUITÉS The abbreviations employed in this work follow those in B. Mathieu, Abréviations des périodiques et collections en usage à l'IFAO (4th ed., Cairo, 2003) and G. Müller, H. Balz and G. Krause (eds), Theologische Realenzyklopädie, vol 26: S. M. Schwertner, Abkürzungsverzeichnis (2nd ed., Berlin - New York, 1994). Presented to NAGUIB KANAWati AM FAHA Professor, Macquarie University, Sydney Member of the Order of Australia Fellow of the Australian Academy of the Humanities by his Colleagues, Friends, and Students CONT E NT S VOLUM E I PR E FA ce ZAHI HAWASS xiii AC KNOWL E DG E M E NT S xv NAGUIB KANAWATI : A LIF E IN EGYPTOLOGY xvii ANN MCFARLANE NAGUIB KANAWATI : A BIBLIOGRAPHY xxvii SUSANNE BINDER , The Title 'Scribe of the Offering Table': Some Observations 1 GILLIAN BOWEN , The Spread of Christianity in Egypt: Archaeological Evidence 15 from Dakhleh and Kharga Oases EDWARD BROVARSKI , The Hare and Oryx Nomes in the First Intermediate 31 Period and Early Middle Kingdom VIVIENNE G. -
The Corrosive Well Waters of Egypt's Western Desert
The Corrosive Well Waters of Egypt's Western Desert GEOLOGICAL SURVEY WATER-SUPPLY PAPER 1757-O Prepared in cooperation with the Arab Republic of Egypt under the auspices of the United States Agency for International Development The Corrosive Well Waters of Egypt's Western Desert By FRANK E. CLARKE CONTRIBUTIONS TO THE HYDROLOGY OF AFRICA AND THE MEDITERRANEAN REGION GEOLOGICAL SURVEY WATER-SUPPLY PAPER 1757-O Prepared in Cooperation with the Arab Republic of Egypt under the auspices of the United States Agency for International Development UNITED STATES GOVERNMENT PRINTING OFFICE, WASHINGTON : 1979 UNITED STATES DEPARTMENT OF THE INTERIOR CECIL D. ANDRUS, Secretary GEOLOGICAL SURVEY H. William Menard, Director Library of Congress Cataloging in Publication Data Clarke, Frank Eldridge, 1913 The corrosive well waters of Egypt's western desert. (Contributions to the hydrology of Africa and the Mediterranean region) (Geological Survey water-supply paper; 1757-0) "Prepared in cooperation with the Arab Republic of Egypt, under the aus pices of the United States Agency for International Development." Bibliography: p. Includes index Supt. of Docs. no. : I 19.16 : 1757-0 1. Corrosion resistant materials. 2. Water, Underground Egypt. 3. Water quality Egypt. 4. Wells Egypt Corrosion. 5. Pumping machinery Cor rosion. I. United States. Agency for International Development. II. Title. III. Series. IV. Series: United States. Geological Survey. Water-supply paper; 1757-0. TA418.75.C58 627'.52 79-607011 For sale by Superintendent of Documents, U.S. Government -
Changing Power Relations in the Nile River Basin: Unilateralism Vs
www.water‐alternatives.org Volume 2 | Issue 2 Cascão, A.E. 2009. Changing power relations in the Nile river basin: Unilateralism vs. cooperation? Water Alternatives 2(2): 245‐268 Changing Power Relations in the Nile River Basin: Unilateralism vs. Cooperation? Ana Elisa Cascão Department of Geography, King’s College of London, United Kingdom; [email protected] ABSTRACT: The aim of this article is to identify where and how power relations in the Nile river basin have changed over the past decade, and to analyse how these dynamics have influenced not only the political relations between upstream and downstream riparians but also the management and allocation of the shared Nile water resources. The article sheds light on the ongoing political and economic changes in the upstream countries (as well as in Sudan) and on how these dynamics might affect and challenge both the regional balance of power and the ongoing regional cooperation process. A critical analysis of the relationship between power shifts and the evolution of the Nile Basin Initiative (NBI) is then provided. Finally, the article questions how unilateralist and multilateralist hydropolitical trends have co‐existed in the Nile basin, and identifies possible future scenarios. KEYWORDS: Nile river basin, power relations, change, unilateralism, cooperation, Egypt, Sudan, Ethiopia INTRODUCTION This first section aims to briefly introduce some key events in Nile basin hydropolitics, which will be important in order to frame the analysis of ongoing power dynamics. Figure 1 shows a time line displaying major events with regional implications, such as agreements, creation of regional water institutions and negotiations. -
Jurassic-Cretaceous Palynomorphs, Palynofacies, and Petroleum
Jurassic-Cretaceous palynomorphs, palynofacies, and petroleum potential of the Sharib-1X and Ghoroud-1X wells, north Western Desert, Egypt Author(s): Zobaa, MK (Zobaa, Mohamed K.)[ 1 ] ; El Beialy, SY (El Beialy, Salah Y.)[ 2 ] ; El- Sheikh, HA (El-Sheikh, Hassan A.)[ 1 ] ; El Beshtawy, MK (El Beshtawy, Mohamed K.)[ 1 ] Source: JOURNAL OF AFRICAN EARTH SCIENCES Volume: 78 Pages: 51-65 DOI: 10.1016/j.jafrearsci.2012.09.010 Published: FEB 2013 Abstract: Palynomorph and palynofacies analyses have been performed on 93 cutting samples from the Jurassic Masajid Formation and Cretaceous Alam El Bueib, Alamein, Dahab, Kharita, and Bahariya formations in the Sharib-1X and Ghoroud-1X wells, north Western Desert, Egypt. Two palynological biozones are proposed for the studied interval of the Sharib-1X well: the Systematophora penicillata-Escharisphaeridia pocockii Assemblage Zone (Middle to Late Jurassic) and the Cretacaeiporites densimurus-Elateroplicites africaensis-Reyrea polymorpha Assemblage Zone (mid-Cretaceous: late Albian to early Cenomanian). Spore coloration and visual kerogen analysis are used to assess the thermal maturation and source rock potential. Mature oil prone to overmature gas prone source rocks occur in the studied interval of the Sharib-1X well, whereas highly mature to overmature gas prone source rocks occur in the studied interval of the Ghoroud-1X well. Palynofacies and palynomorph assemblages in both wells reflect shallow marine conditions throughout the Jurassic and the late Albian and early Cenomanian. During these times, warm and dry climatic conditions prevailed. The Cretaceous palynomorph assemblages of the Sharib-IX well correlate with the Albian-Cenomanian Elaterates Province of Herngreen et al. -
Survey of the Most Common Insect Species on Some Foraging Crops of Honeybees in Dakhla Oasis, New Valley Governorate, Egypt
J. Eco. Heal. Env. 5, No. 1, 35-40 (2017) 35 Journal of Ecology of Health & Environment An International Journal http://dx.doi.org/10.18576/jehe/050105 Survey of the Most Common Insect Species on Some Foraging Crops of Honeybees in Dakhla Oasis, New Valley Governorate, Egypt Mahmoud S. O. Mabrouk1,, and Mohamed Abdel - Moez Mahbob2,* 1 Bee keeping Res. Dept. Plant Protection Res. Institute, A.R. C., Egypt. 2 Department of Zoology & Entomology, Faculty of Science, New Valley Branch, Assiut University, New Valley, Egypt. Received: 21 Feb. 2016, Revised: 22 Mar. 2016, Accepted: 24 Mar. 2016. Published online: 1 Jan. 2017. Abstract: When studying the presence of beneficial insects and harmful on each alfalfa, Egyptian clover and faba been fields at the New Valley Governorate, Egypt, it turned out to include 46 species belonging to 33 family that follow 9 orders divided in to three groups (pests – natural enemies – pollinators). The study result also showed that the largest number of species of insects recorded on the crop fields under study belong to the order Hymenoptera where 19 species belonging 12 families. On the other hand, a total of pollinators has ranked first in the number of insect species that have been counted during the experimental crops in this study, and the main pollinators of those crops in Dakhla Oasis, New Valley Governorate, were honeybees. Keywords: Honeybees, alfalfa, faba been, pests, pollinators reported from alfalfa in the US, with perhap100-150 of 1 Introduction these causing some degree of injury. Few of these, however, can be described as key pest species, the rest are Pollinators playing a big role of pollination specially in the of only local or sporadic importance, or are incidental cross pollination crops and increased the feddan production herbivores, intomophagous (parasites and predators), or of seeds. -
Palynology of Some Cretaceous Mudstones from Southeast Aswan, Egypt: Significance to Regional Stratigraphy
Journal of African Earth Sciences 47 (2007) 1–8 www.elsevier.com/locate/jafrearsci Palynology of some Cretaceous mudstones from southeast Aswan, Egypt: significance to regional stratigraphy Magdy S. Mahmoud *, Mahmoud A. Essa Geology Department, Faculty of Science, Assiut University, Assiut 71516, Egypt Received 2 February 2006; received in revised form 5 October 2006; accepted 18 October 2006 Available online 30 November 2006 Abstract The basal mudstones from the El-Nom borehole in the Gebel Abraq area in southern Egypt have yielded a diverse and relatively well preserved terrestrial palynoflora that includes Balmeisporites holodictyus, Crybelosporites pannuceus, Foveotricolpites gigantoreticulatus, Nyssapollenites albertensis, Retimonocolpites variplicatus and Rousea delicipollis. These suggest an Albian–Cenomanian age and deposi- tion in a fluvio-deltaic environment; no marine phytoplankton is reported. The fern-dominated palynoflora and the overwhelming pres- ence of kaolinitic clays suggest a warm, humid palaeoclimate. According to available knowledge, the mudstones in the Gebel Abraq area, equivalents of the so-called ‘‘Timsah Formation’’, might be correlated with an older rock unit, the Maghrabi Formation, based on the new palynological age assessment. This new definition of local stratigraphy implies that the Bernice sheet of geological map of Egypt [Klitzsch, E., List, F., Po¨hlmann, G., 1987. Geological map of Egypt, sheet NF 36 NE Bernice, 1: 500000. Conoco and the Egyptian General Petroleum Corporation, Cairo] ought to be reconsidered. Ó 2006 Elsevier Ltd. All rights reserved. Keywords: Terrestrial palynology; Stratigraphy; Cretaceous; Egypt 1. Introduction and geological setting The siliciclastics of the ‘‘Nubian Sandstone’’ rocks are of predominantly continental origin. They are widely exposed This study was conducted as part of a sustainable devel- in central and southern Egypt, and are mostly Cretaceous in opment project in southern Egypt in a trial to improve the age. -
Morphologic Characteristics and Migration Rate Assessment of Barchan Dunes in the Southeastern Western Desert of Egypt
Geomorphology 257 (2016) 57–74 Contents lists available at ScienceDirect Geomorphology journal homepage: www.elsevier.com/locate/geomorph Morphologic characteristics and migration rate assessment of barchan dunes in the Southeastern Western Desert of Egypt M.A. Hamdan, A.A. Refaat ⁎, M. Abdel Wahed Geology Department, Faculty of Science, Cairo University, Giza, Egypt article info abstract Article history: This work explores the morphologic characteristics of aeolian dune sand in the southeastern part of Western De- Received 3 May 2015 sert of Egypt. It aims to assess the movement of barchan dunes and evaluate their environmental influence on the Received in revised form 6 December 2015 Toshka Project. Morphometric investigation of barchan dunes in the Toshka area revealed that most barchans Accepted 30 December 2015 have high length/width (a/c) ratios (fat to pudgy), while one-fifth of the studied barchans have lower a/c ratios Available online 31 December 2015 and so appear normal in their morphologic forms. Statistical analysis of the main parameters of barchan dunes in Key words: Toshka and other desert regions in the Kharga (Egypt), Kuwait, Southern Morocco, California and Southern Peru Barchan dune demonstrates that barchans of the Toshka area are distinctive in their appearance. They are characterized by dis- dune migration tinct aspect with higher values of length and width and greater growth in height. The high-energy wind environ- dune encroachment hazard ment in addition to the large amount of drifting sand are principal factors responsible for the unique shape of Western Desert of Egypt Toshka barchans. The migration rate of barchan dunes in four chosen test locations, within the central and western Toshka area, ranges from about 3 to 10.82 m/year. -
MOST ANCIENT EGYPT Oi.Uchicago.Edu Oi.Uchicago.Edu
oi.uchicago.edu MOST ANCIENT EGYPT oi.uchicago.edu oi.uchicago.edu Internet publication of this work was made possible with the generous support of Misty and Lewis Gruber MOST ANCIE NT EGYPT William C. Hayes EDITED BY KEITH C. SEELE THE UNIVERSITY OF CHICAGO PRESS CHICAGO & LONDON oi.uchicago.edu Library of Congress Catalog Card Number: 65-17294 THE UNIVERSITY OF CHICAGO PRESS, CHICAGO & LONDON The University of Toronto Press, Toronto 5, Canada © 1964, 1965 by The University of Chicago. All rights reserved. Published 1965. Printed in the United States of America oi.uchicago.edu WILLIAM CHRISTOPHER HAYES 1903-1963 oi.uchicago.edu oi.uchicago.edu INTRODUCTION WILLIAM CHRISTOPHER HAYES was on the day of his premature death on July 10, 1963 the unrivaled chief of American Egyptologists. Though only sixty years of age, he had published eight books and two book-length articles, four chapters of the new revised edition of the Cambridge Ancient History, thirty-six other articles, and numerous book reviews. He had also served for nine years in Egypt on expeditions of the Metropolitan Museum of Art, the institution to which he devoted his entire career, and more than four years in the United States Navy in World War II, during which he was wounded in action-both periods when scientific writing fell into the background of his activity. He was presented by the President of the United States with the bronze star medal and cited "for meritorious achievement as Commanding Officer of the U.S.S. VIGILANCE ... in the efficient and expeditious sweeping of several hostile mine fields.., and contributing materially to the successful clearing of approaches to Okinawa for our in- vasion forces." Hayes' original intention was to work in the field of medieval arche- ology. -
Paranoia on the Nile
The politics of flood insecurity Framing contested river management projects Jeroen F. Warner Promotoren: Prof. Dr. Ir. D.J.M. Hilhorst Hoogleraar Humanitaire Hulp en Wederopbouw Prof. Dr. Ir. C. Leeuwis Hoogleraar Communicatie en Innovatie Studies Promotiecommissie Prof. Dr. J.A. Allan King‟s College, London Prof. Dr. H.J.M. Goverde Wageningen Universiteit / Radboud Universiteit Nijmegen Prof. Dr. Mr. B.J.M. van der Meulen Wageningen Universiteit Prof. Dr. J.H. de Wilde Rijksuniversiteit Groningen Dit onderzoek is uitgevoerd binnen de onderzoeksschool CERES – Research School for Resource Studies for Development. The politics of flood insecurity Framing contested river management projects Jeroen F. Warner Proefschrift ter verkrijging van de graad van doctor op gezag van de rector magnificus van Wageningen Universiteit, prof. dr. M.J. Kropff, in het openbaar te verdedigen op dinsdag 18 maart 2008 des namiddags om 16.00 uur in de Aula. Jeroen F. Warner The politics of flood insecurity Framing contested river management projects ISBN 978-80-8504-897-8 Table of Contents List of Figures, Tables and Boxes List of Abbreviations 1. Introduction: The politics of floods and fear 1 2. Midnight at Noon? The dispute over Toshka, Egypt 31 3. Resisting the Turkish pax aquarum? The Ilısu Dam dispute as a multi-level struggle 57 4. Turkey and Egypt – tales of war, peace and hegemony 83 5. Death of the mega-projects? The controversy over Flood Action Plan 20, Bangladesh 111 6. The Maaswerken project: Fixing a hole? 145 7. Public Participation in emergency flood storage in the Ooij polder – a bridge too far? 173 8. -
Archaeology on Egypt's Edge
doi: 10.2143/AWE.12.0.2994445 AWE 12 (2013) 117-156 ARCHAEOLOGY ON EGYPT’S EDGE: ARCHAEOLOGICAL RESEARCH IN THE DAKHLEH OASIS, 1819–1977 ANNA LUCILLE BOOZER Abstract This article provides the first substantial survey of early archaeological research in Egypt’s Dakhleh Oasis. In addition to providing a much-needed survey of research, this study embeds Dakhleh’s regional research history within a broader archaeological research framework. Moreover, it explores the impact of contemporaneous historical events in Egypt and Europe upon the development of archaeology in Dakhleh. This contextualised approach allows us to trace influences upon past research trends and their impacts upon current research and approaches, as well as suggest directions for future research. Introduction This article explores the early archaeological research in Egypt’s Dakhleh Oasis within the framework of broad archaeological trends and contemporaneous his- torical events. Egypt’s Western Desert offered a more extreme research environ- ment than the Nile valley and, as a result, experienced a research trajectory different from and significantly later than most of Egyptian archaeology. In more recent years, the archaeology along Egypt’s fringes has provided a significant contribution to our understanding of post-Pharaonic Egypt and it is important to understand how this research developed.1 The present work recounts the his- tory of research in Egypt’s Western Desert in order to embed the regional research history of the Dakhleh Oasis within broader trends in Egyptology, archaeology and world historical events in Egypt and Europe (Figs. 1–2).2 1 In particular, the western oases have dramatically reshaped our sense of the post-Pharaonic occupation of Egypt as well as the ways in which the Roman empire interfaced with local popula- tions. -
An Oasis City 1. Trimithis
An Oasis City 1. Trimithis: The city and its gods [opening slide] The subject of these three lectures is a city in a part of the western desert of Egypt that in ancient times was considered the western or Inner part of the Great Oasis and is today the Dakhla Oasis. Then the city was called Trimithis; today the site is called Amheida. It has been the subject of a field project that I have directed during the past ten years, with seven years of excavation to date. We owe almost all of our knowledge of Trimithis to archaeology and to documents discovered through excavation, both at the site itself and from the work of the Dakhleh Oasis Project at Kellis, the archaeological site of Ismant el-Kharab, since 1986. The decision to begin a field project at Amheida had multiple reasons. Among them was a sense, shared with some other papyrologists, and eloquently articulated by Claudio Gallazzi in 1992 at the Copenhagen congress of papyrology, that our generation might be the last one with significant opportunities to find ancient texts written in ink on papyrus, pottery, and wood in Egypt, where settlement expansion and the rising ground-water levels that are the result of the High Dam and agricultural development and population growth more generally are rapidly destroying the dry conditions that preserved hundreds of thousands of papyri and ostraka until the last century. Another motive was a more general assessment that there are few high-quality sustained projects in the archaeology of Graeco-Roman Egypt, especially linking texts to their archaeological contexts, and more would be beneficial to the development of a more archaeologically grounded history of this society; a third motive was the need for Columbia 1 University, where I was then teaching, to have a means for teaching archaeological fieldwork methods to its students. -
Plant Diversity Around Springs and Wells in Five Oases of the Western Desert, Egypt
INTERNATIONAL JOURNAL OF AGRICULTURE & BIOLOGY 1560–8530/2006/08–2–249–255 http://www.fspublishers.org Plant Diversity Around Springs and Wells in Five Oases of the Western Desert, Egypt MONIER M. ABD EL-GHANI1 AND AHMED M. FAWZY† The Herbarium, Faculty of Science, Cairo University, Giza 12613, Egypt †The Herbarium, Flora and Phyto-Taxonomy Research, Horticultural Research Institute, Agricultural Research Centre, Dokki, Giza, Egypt 1Corresponding author’s e-mail: [email protected] ABSTRACT This study was conducted to analyse the floristic composition around the wells and springs in five oases (Siwa, Bahariya, Farafra, Dakhla & Kharga) of the Western Desert of Egypt in terms of habitat and species diversity. A total 59 sites were surveyed and distributed as follows: twelve in Siwa, fifteen in Bahariya, twelve in Farafra, eight in Dakhla and twelve in Kharga Oasis. Altogether, 172 species (131 genera & 39 families) of the vascular plants were recorded from the five main distinguished habitats, viz., farmlands (H1), canal banks (H2), reclaimed lands (H3), waste lands (H4) and water bodies (H5). The most diversified habitats with high species richness were the farmlands and the canal banks, whereas the least diversified was the water bodies. The ancient irrigation pattern in these oases were studied and described. Bahariya Oasis was the richest in species followed by Siwa, while the lowest number of species was found in Dakhla Oasis, which represents the least affected area by the anthropogenic activities. Forty-three species or 25.1% of the total recorded flora confined to a certain study area: 3 in Siwa, 29 in Bahariya, 3 in Farafra and 8 in Kharga Oasis.