Nitrenes As Intermediates in the Thermal Decomposition of Aliphatic Azides
Total Page:16
File Type:pdf, Size:1020Kb
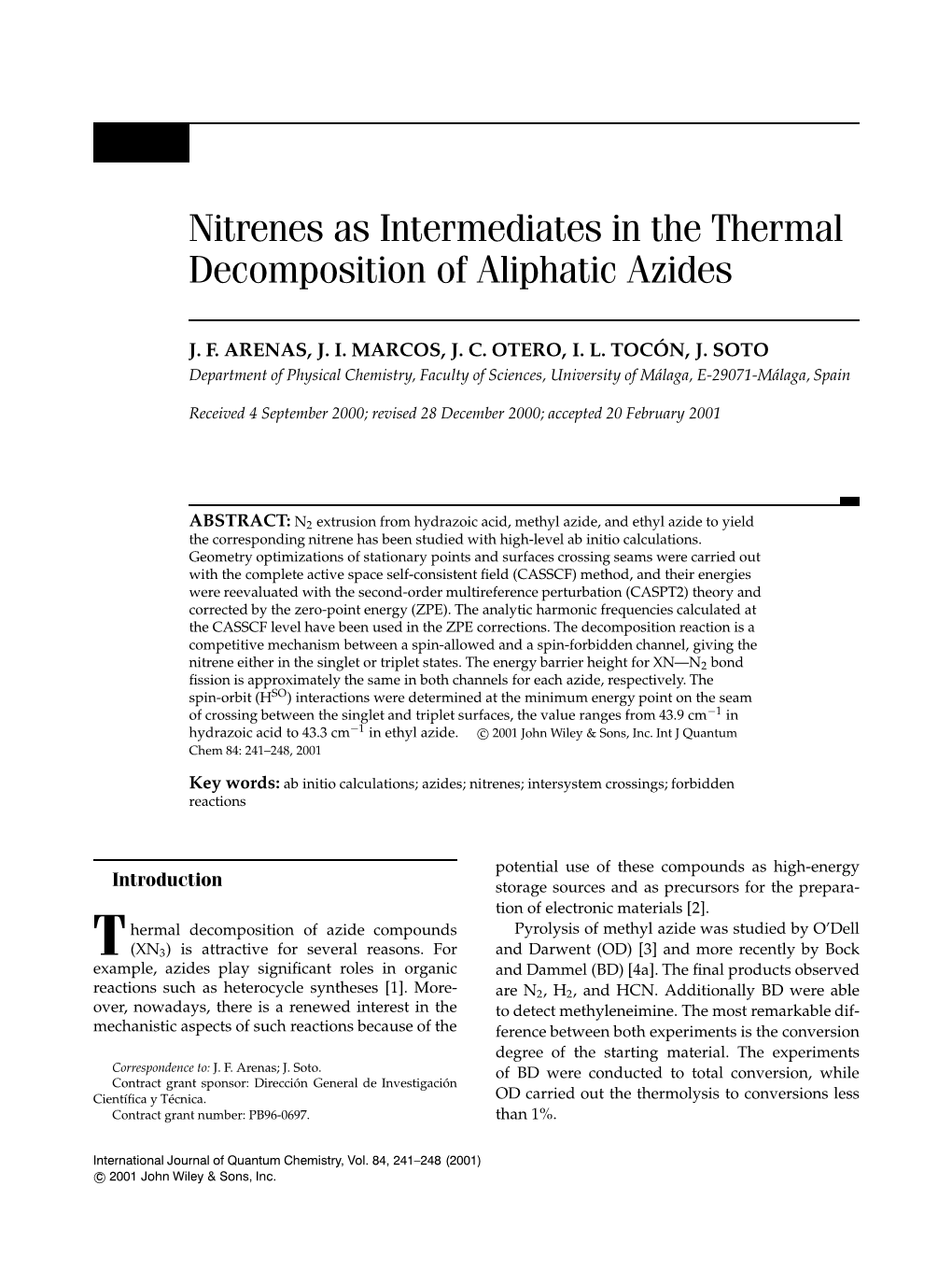
Load more
Recommended publications
-
All-In-One Light-Tunable Borated Phosphors with Chemical And
Research Article www.acsami.org All-In-One Light-Tunable Borated Phosphors with Chemical and Luminescence Dynamical Control Resolution † ‡ § ‡ ∥ ⊥ Chun Che Lin, Yun-Ping Liu, Zhi Ren Xiao, Yin-Kuo Wang, , Bing-Ming Cheng, † # ○ and Ru-Shi Liu*, , , † Department of Chemistry, National Taiwan University, Taipei 106, Taiwan ‡ Department of Physics, National Taiwan Normal University, Taipei 106, Taiwan § Graduate Institute of Applied Physics, National Chengchi University, Taipei 116, Taiwan ∥ Center for General Education and Department of Physics, National Taiwan Normal University, Taipei 106, Taiwan ⊥ National Synchrotron Radiation Research Center, Hsinchu 300, Taiwan # Department of Mechanical Engineering, National Taipei University of Technology, Taipei 10608, Taiwan ○ Graduate Institute of Manufacturing Technology, National Taipei University of Technology, Taipei 10608, Taiwan *S Supporting Information ABSTRACT: Single-composition white-emitting phosphors with supe- rior intrinsic properties upon excitation by ultraviolet light-emitting diodes are important constituents of next-generation light sources. 3+ 3+ Borate-based phosphors, such as NaSrBO3:Ce and NaCaBO3:Ce , have stronger absorptions in the near-ultraviolet region as well as better chemical/physical stability than oxides. Energy transfer effects from sensitizer to activator caused by rare-earth ions are mainly found in the obtained photoluminescence spectra and lifetime. The interactive mechanisms of multiple dopants are ambiguous in most cases. We 3+ 3+ adjust the doping concentration in NaSrBO3:RE (RE = Ce ,Tb , Mn2+) to study the energy transfer effects of Ce3+ to Tb3+ and Mn2+ by comparing the experimental data and theoretical calculation. The vacuum-ultraviolet experimental determination of the electronic energy levels for Ce3+ and Tb3+ in the borate host regarding the 4f−5d and 4f− 4f configurations are described. -
Powder Processing, Densification Behaviour, Microstructure and Mechanical Properties Of
POWDER PROCESSING, DENSIFICATION BEHAVIOUR, MICROSTRUCTURE AND MECHANICAL PROPERTIES OF AL2O3- 50 Vol% ZrO2 COMPOSITES A Thesis Submitted in Partial Fulfillment of the Requirements for the Degree of DOCTOR OF PHILOSOPHY in ENGINEERING by RAGHUNATH PRASAD RANA (Roll No: 50408003) Supervisors: Dr. Santanu Bhattacharyya Dr. Swadesh Kumar Pratihar DEPARTMENT OF CERAMIC ENGINEERING NATIONAL INSTITUTE OF TECHNOLOGY ROURKELA NOVEMBER, 2009 NATIONAL INSTITUTE OF TECHNOLOGY ROURKELA, INDIA CERTIFICATE This is to certify that the thesis entitled “Powder Processing, Densification Behaviour, Microstructure and Mechanical Properties of Al2O3- 50 vol% ZrO2 Composites” being submitted by Mr. Raghunath Prasad Rana for the degree of Doctor of Philosophy in Engineering, is a record of bonafied research work carried out by him under our supervision at Department of Ceramic Engineering. To the best of our knowledge, the work has not been submitted to any other University or Institute for the award of any degree or diploma. (S Bhattacharyya) (S K Pratihar) Professor Associate Professor Department of Ceramic Engineering Department of Ceramic Engineering Dedicated to my parents CONTENTS Page No. Abstract i Acknowledgement iv List of Figures vi List of Tables xii Chapter I - Introduction Introduction 1 References 12 Chapter II - Literature Review 2.1 Transformation Toughening Mechanism 15 2.2 Transformation Toughening Models 17 2.3 Other Toughening Mechanisms for ZTA Ceramics 21 2.3.1 Toughening by Crack Deflection 21 2.3.2 Toughening by the Generation of Compressive -
Oxygen and Proton Reduction by Decamethylferrocene in Non-Aqueous Acidic Mediaw
View Article Online / Journal Homepage / Table of Contents for this issue COMMUNICATION www.rsc.org/chemcomm | ChemComm Oxygen and proton reduction by decamethylferrocene in non-aqueous acidic mediaw Bin Su,za Imren Hatay,ab Pei Yu Ge,a Manuel Mendez,a Clemence Corminboeuf,c Zdenek Samec,d Mustafa Ersozb and Hubert H. Girault*a Received (in Cambridge, UK) 18th January 2010, Accepted 3rd March 2010 First published as an Advance Article on the web 18th March 2010 DOI: 10.1039/b926963k Experimental studies and density functional theory (DFT) yellow, to which addition of an acid, hydrogen tetrakis(penta- computations suggest that oxygen and proton reduction by fluorophenyl)borate (HTB),y led to an immediate colour decamethylferrocene (DMFc) in 1,2-dichloroethane involves change to bright green. In the UV-visible spectrum, the protonated DMFc, DMFcH+, as an active intermediate species, absorption band of DMFc centered at 425 nm is replaced by producing hydrogen peroxide and hydrogen in aerobic and a strong band of DMFc+ at 779 nm. Moreover, by shaking anaerobic conditions, respectively. the green organic solution with pure water and then by titrating this aqueous phase with sodium iodide (NaI), O2 reduction by ferrocene and its derivatives in organic media triiodide was detected with a spectroscopic signature at 286 nm in the presence of an acid, such as trichloroacetic and trifluoro- and 330 nm. Triiodide was generated by oxidation of iodide by 7 acetic acids and perchloric acid, has been known for many H2O2. These results suggest the occurrence of oxygen 1–5 years. Recently, O2 reduction has also been studied in reduction to H2O2 by DMFc in DCE in the presence of the biphasic systems composed of an organic solvent containing organic acid: a ferrocene derivative in contact with an aqueous inorganic 6–8 + À - + À acid solution containing a very lipophilic anion. -
Wo 2010/138154 A2
(12) INTERNATIONAL APPLICATION PUBLISHED UNDER THE PATENT COOPERATION TREATY (PCT) (19) World Intellectual Property Organization International Bureau (10) International Publication Number (43) International Publication Date 2 December 2010 (02.12.2010) WO 2010/138154 A2 (51) International Patent Classification: HN, HR, HU, ID, IL, IN, IS, JP, KE, KG, KM, KN, KP, AOlG 15/00 (2006.01) KR, KZ, LA, LC, LK, LR, LS, LT, LU, LY, MA, MD, ME, MG, MK, MN, MW, MX, MY, MZ, NA, NG, NI, (21) International Application Number: NO, NZ, OM, PE, PG, PH, PL, PT, RO, RS, RU, SC, SD, PCT/US2010/001379 SE, SG, SK, SL, SM, ST, SV, SY, TH, TJ, TM, TN, TR, (22) International Filing Date: TT, TZ, UA, UG, US, UZ, VC, VN, ZA, ZM, ZW. 10 May 2010 (10.05.2010) (84) Designated States (unless otherwise indicated, for every (25) Filing Language: English kind of regional protection available): ARIPO (BW, GH, GM, KE, LR, LS, MW, MZ, NA, SD, SL, SZ, TZ, UG, (26) Publication Language: English ZM, ZW), Eurasian (AM, AZ, BY, KG, KZ, MD, RU, TJ, (30) Priority Data: TM), European (AL, AT, BE, BG, CH, CY, CZ, DE, DK, 12/453,444 12 May 2009 (12.05.2009) US EE, ES, FI, FR, GB, GR, HR, HU, IE, IS, IT, LT, LU, PCT/US 10/00 188 25 January 20 10 (25 .0 1.20 10) US LV, MC, MK, MT, NL, NO, PL, PT, RO, SE, SI, SK, PCT/US 10/00 187 25 January 20 10 (25 .0 1.20 10) US SM, TR), OAPI (BF, BJ, CF, CG, CI, CM, GA, GN, GQ, GW, ML, MR, NE, SN, TD, TG). -
An Exploration of the C9H8O Energy Surface in the Vicinity of Bicyclo[5.2.0]Nona-2,5,8-Trien-4-One David Michael Lokensgard Iowa State University
Iowa State University Capstones, Theses and Retrospective Theses and Dissertations Dissertations 1978 An exploration of the C9H8O energy surface in the vicinity of bicyclo[5.2.0]nona-2,5,8-trien-4-one David Michael Lokensgard Iowa State University Follow this and additional works at: https://lib.dr.iastate.edu/rtd Part of the Organic Chemistry Commons Recommended Citation Lokensgard, David Michael, "An exploration of the C9H8O energy surface in the vicinity of bicyclo[5.2.0]nona-2,5,8-trien-4-one " (1978). Retrospective Theses and Dissertations. 6465. https://lib.dr.iastate.edu/rtd/6465 This Dissertation is brought to you for free and open access by the Iowa State University Capstones, Theses and Dissertations at Iowa State University Digital Repository. It has been accepted for inclusion in Retrospective Theses and Dissertations by an authorized administrator of Iowa State University Digital Repository. For more information, please contact [email protected]. INFORMATION TO USERS This material was produced from a microfilm copy of the original document. While the most advanced technological means to photograph and reproduce this document have been used, the quality is heavily dependent upon the quality of the original submitted. The following explanation of techniques is provided to help you understand markings or patterns which may appear on this reproduction. 1. The sign or "target" for pages apparently lacking from the document photographed is "Missing Page(s)". If it was possible to obtain the missing page(s) or section, they are spliced into the film along with adjacent pages. This may have necessitated cutting thru an image and duplicating adjacent pages to insure you complete continuity. -
1 Introduction
Synthesis, characterization and photocatalytic performances of p-type Carbon Nitride Gabriele Capilli, Mattia Costamagna, Fabrizio Sordello, Claudio Minero * Dipartimento di Chimica, Università degli Studi di Torino – via Pietro Giuria 5 -10125 Torino, Italy ABSTRACT p-Type organic semiconductors are attractive to develop new photo- and photoelectro-catalytic systems, particularly if the conduction band is located at negative redox potentials. An easy synthetic route to produce carbon-doped carbon nitride (Cn>3N4) with a stable p-type semiconducting character was optimized. The precursors used were melamine and triaminopyrimidine. The p-type C>3N4 exhibited a remarkable photoactivity under visible light compared with pristine C3N4, which is a stable n-type organic semiconductor deeply studied as heterogeneous photocatalyst for many environmental applications. The photoelectrochemical features of the synthesized p-type materials and pristine C3N4 were deeply investigated with chronopotentiometry and cyclic voltammetry, in the dark and under different illumination conditions, and then compared with the photocatalytic activity using 2- fluorophenol as substrate and different radiation sources. The p-doping with carbon of C3N4 reduces the band gap, slightly moves the potential of the valence band, and increases the conduction band to more positive potentials, thus precluding the application of these materials when large negative redox potentials are needed. KEYWORDS. p-doped carbon nitride, visible light catalyst, p-type semiconductor, heterogeneous -
Primordial Pairing and Binding of Superheavy Charged Particles in the Early Universe¦ V
JETP Letters, Vol. 77, No. 7, 2003, pp. 335–338. From Pis’ma v Zhurnal Éksperimental’noÏ i TeoreticheskoÏ Fiziki, Vol. 77, No. 7, 2003, pp. 403–406. Original English Text Copyright © 2003 by Dubrovich, Khlopov. Primordial Pairing and Binding of Superheavy Charged Particles in the Early Universe¶ V. K. Dubrovich*, 1 and M. Yu. Khlopov**, ***, **** * Special Astrophysical Observatory, Russian Academy of Sciences, St. Petersburg, 196140 Russia 1 e-mail:[email protected] ** Keldysh Institute of Applied Mathematics, Russian Academy of Sciences, Moscow, 125047 Russia *** Moscow Engineering Physics Institute, Moscow, 115409 Russia **** Physics Department, Università degli studi “La Sapienza,” I 00185 Roma, Italy Received July 10, 2002; in final form, February 18, 2003 Primordial superheavy particles, considered as the source of Ultra High Energy Cosmic Rays (UHECR) and produced in local processes in the early Universe, should bear some strictly or approximately conserved charge to be sufficiently stable to survive to the present time. Charge conservation dictates that they be produced in pairs, and the estimated separation of particle and antiparticle in such a pair is shown to be in some cases much smaller than the average separation determined by the averaged number density of considered particles. If the new U(1) charge is the source of a long-range field similar to the electromagnetic field, the particle and antipar- ticle, possessing that charge, can form a primordial bound system with an annihilation timescale, which can satisfy the conditions assumed for this type of UHECR source. These conditions severely constrain the possible properties of the considered particles. © 2003 MAIK “Nauka/Interperiodica”. -
Reverberation Mapping of the Dusty Torus in Active Galactic Nuclei
Reverberation Mapping of the Dusty Torus in Active Galactic Nuclei by Erich Franz Geisert Bachelor of Science Astronomy and Astrophysics Florida Institute of Technology 2011 A thesis submitted to the College of Sciences at Florida Institute of Technology in partial fulfillment of the requirements for the degree of Master of Science in Space Sciences Melbourne, Florida April 2016 c Copyright 2016 Erich Franz Geisert All Rights Reserved The author grants permission to make single copies We the undersigned committee hereby recommend that the attached document be accepted as fulfilling in part the requirements for the degree of Master of Science in Space Sciences. \Reverberation Mapping of the Dusty Torus in Active Galactic Nuclei", a thesis by Erich Franz Geisert Daniel Batcheldor, Ph.D. Associate Professor, Physics and Space Sciences Major Advisor Eric Perlman, Ph.D. Professor, Physics and Space Sciences Committee Member V´eroniquePetit, Ph.D. Assistant Professor, Physics and Space Sciences Committee Member Isaac Silver, Ph.D. Assistant Professor, College of Aeronautics External Committee Member Daniel Batcheldor, Ph.D. Interim Department Head, Physics and Space Sciences Abstract TITLE: Reverberation Mapping of the Dusty Torus in Active Galactic Nuclei AUTHOR: Erich Franz Geisert MAJOR ADVISOR: Daniel Batcheldor, Ph.D. The Dusty Torus is a structure that is believed to exist in Active Galactic Nu- clei. The Torus is physically too small to be spatially resolved, so Reverberation Mapping can be performed in order to replace spatial resolution with tempo- ral resolution, yielding observations that can unravel the mystery of the Dusty Torus. One of the unknowns about the Torus is how the dust is distributed. -
Failure Modes of Electronics
First Edition, 2011 ISBN 978-93-81157-19-0 © All rights reserved. Published by: The English Press 4735/22 Prakashdeep Bldg, Ansari Road, Darya Ganj, Delhi - 110002 Email: [email protected] Table of Contents Chapter 1- Failure Modes of Electronics Chapter 2 - Electromigration Chapter 3 - Electrostatic discharge, Overvoltage & Overcurrent Chapter 4 - Semiconductor-Related Failures Chapter 5 - Stress-Related Failures Chapter 6 - Optoelectronics Failures Chapter 7 - Microelectromechanical Systems Failures Chapter 8 - Vacuum Tubes Failures Chapter- 1 Failure Modes of Electronics Electronic devices have a wide range of failure modes. These can be distinguished by their development in time (sudden failure or gradual degradation), by environmental effects (e.g. corrosion, ionizing radiation) or by the electrical parameter which was exceeded (e.g. electrostatic discharge, overvoltage, overcurrent, etc.). Failures most commonly occur at the beginning and near the end of the lifetime of the parts. Burn-in procedures are used to detect early failures. Presence of parasitic structures, irrelevant for normal operation, may become important in the context of failures; such structures can be both a source of failure and a protective device. A sudden fail-open failure can cause multiple secondary failures, when the event is fast and the circuit contains an inductance. The suddenly interrupted current flow in combination with the inductance then causes large voltage spikes, which for very fast events may exceed 500 volts. A burned metallization on a chip may then cause secondary overvoltage damage. Packaging-related failures Electronic packaging, acting as the barrier between the materials of the electronic parts and the environment, is very susceptible to environmental factors. -
The Nonhuman Turn Center for 21St Century Studies Richard Grusin, Series Editor 0 the Nonhuman
The Nonhuman Turn Center for 21st Century Studies Richard Grusin, Series Editor 0 The Nonhuman Turn0 Richard Grusin, Editor Center for 21st Century Studies University of Minnesota Press Minneapolis • London An earlier version of chapter 6 was published as Wendy Hui Kyong Chun, “Crisis, Crisis, Crisis, or Sovereignty and Networks,” Theory, Culture, and Society 28, no. 6 (2011): 91–112; reprinted by permission of SAGE Publications, Ltd., London, Los Angeles, New Delhi, Singapore, and Washington, D.C.; copyright 2011 Theory, Culture, and Society, SAGE Publications. An earlier version of chapter 9 was published as Jane Bennett, “Systems and Things: A Response to Graham Harman and Timothy Morton,” New Literary History 43, no. 2 (Spring 2012): 225–33; copyright 2012 New Literary History, University of Virginia; reprinted with permission of the Johns Hopkins University Press. Copyright 2015 by the Board of Regents of the University of Wisconsin System All rights reserved. No part of this publication may be reproduced, stored in a retrieval system, or transmitted, in any form or by any means, electronic, mechanical, photocopying, recording, or otherwise, without the prior written permission of the publisher. Published by the University of Minnesota Press 111 Third Avenue South, Suite 290 Minneapolis, MN 55401- 2520 http://www.upress.umn.edu Library of Congress Cataloging-in-Publication Data The nonhuman turn / Richard Grusin, editor, Center for 21st Century Studies. (21st century studies) Includes bibliographical references and index. ISBN 978-0-8166-9466-2 (hc : alk. paper) ISBN 978-0-8166-9467-9 (pb : alk. paper) 1. Panpsychism—Congresses. 2. Consciousness—Congresses. I. Grusin, Richard A., editor. -
Estudio Computacional a Nivel Dft De La Descomposición Del Cátodo De Una Batería
POR TADA Universidad de Concepción Dirección de Postgrado Facultad de Ingeniería - Programa de Magíster en Ciencias de la Ingeniería con mención en Ingeniería Química ESTUDIO COMPUTACIONAL A NIVEL DFT DE LA DESCOMPOSICIÓN DEL CÁTODO DE UNA BATERÍA APRÓTICA DE Li-O2 Tesis para optar al grado de Magíster en Ciencias de la Ingeniería con mención en Ingeniería Química ADOLFO JOSÉ ANÍBAL SALGADO CASANOVA CONCEPCIÓN-CHILE 2017 Profesor Guía: Ljubisa R. Radovic Naumov Dpto. de Ingeniería Química, Facultad de Ingeniería Universidad de Concepción Se autoriza la reproducción total o parcial, con fines académicos, por cualquier medio o procedimiento, incluyendo la cita bibliográfica del documento. Además, se deja expresamente establecido que las figuras no referenciadas en este documento son de elaboración propia del autor, por lo que no se infringen derechos de autor de terceros. ii AGRADECIMIENTOS Agradezco al departamento de Ingeniería Química, a sus profesores y personal, por los distintos tipos de apoyo que he recibido durante mi magíster, en especial agradezco al profesor Romel Jiménez por su constante entusiasmo y por motivarme a que siguiera mis estudios de postgrado. Agradezco profundamente al profesor Radovic, mi tutor durante esta tesis y también durante mi pregrado. Por ser como es, un apasionado por la ciencia, a veces incomprendido, que hace lo que le gusta: investigar. Por ser tan generoso, tanto en la vida académica, permitiendo participar de múltiples congresos internacionales, como en lo personal, al invitarnos a mí y a otros colegas/estudiantes tantas veces al Latitud Sur. Y por motivarme y enseñarme esos valores que se han perdido un poco en el mundo académico moderno. -
Reactions of State-Selected Atomic Oxygen Ions O+(4S, 2D, 2P) With
Reactions of State-Selected Atomic Oxygen Ions O+(4S, 2D, 2P) with Methane Barbara Cunha de Miranda, Claire Romanzin, Simon Chefdeville, Véronique Vuitton, Ján Žabka, Miroslav Polášek, Christian Alcaraz To cite this version: Barbara Cunha de Miranda, Claire Romanzin, Simon Chefdeville, Véronique Vuitton, Ján Žabka, et al.. Reactions of State-Selected Atomic Oxygen Ions O+(4S, 2D, 2P) with Methane. Journal of Physical Chemistry A, American Chemical Society, 2015, 119 (23), pp.6082-6098. 10.1021/jp512846v. hal-02319338 HAL Id: hal-02319338 https://hal.archives-ouvertes.fr/hal-02319338 Submitted on 13 Jul 2021 HAL is a multi-disciplinary open access L’archive ouverte pluridisciplinaire HAL, est archive for the deposit and dissemination of sci- destinée au dépôt et à la diffusion de documents entific research documents, whether they are pub- scientifiques de niveau recherche, publiés ou non, lished or not. The documents may come from émanant des établissements d’enseignement et de teaching and research institutions in France or recherche français ou étrangers, des laboratoires abroad, or from public or private research centers. publics ou privés. Reactions of State-Selected Atomic Oxygen Ions O+(4S, 2D, 2P) with Methane Barbara Cunha de Miranda1,2,3,#, Claire Romanzin1, Simon Chefdeville1, Véronique Vuitton4, Jan Žabka5, Miroslav Polášek5, and Christian Alcaraz1,3,* 1 Laboratoire de Chimie Physique, UMR 8000 CNRS - Univ. Paris Sud, Bât. 350, FR-91405 Orsay Cedex, France 2 Laboratório de Espectroscopia e Laser, Instituto de Física, Universidade Federal Fluminense, Av. Gal. Milton Tavares de Souza, Boa Viagem, Niterói, RJ, BR-24210-340 Brazil 3 Synchrotron SOLEIL, L'Orme des merisiers, BP 48, St Aubin, FR-91192 Gif sur Yvette, France 4 Univ.