Anomalous Em Signals And
Total Page:16
File Type:pdf, Size:1020Kb
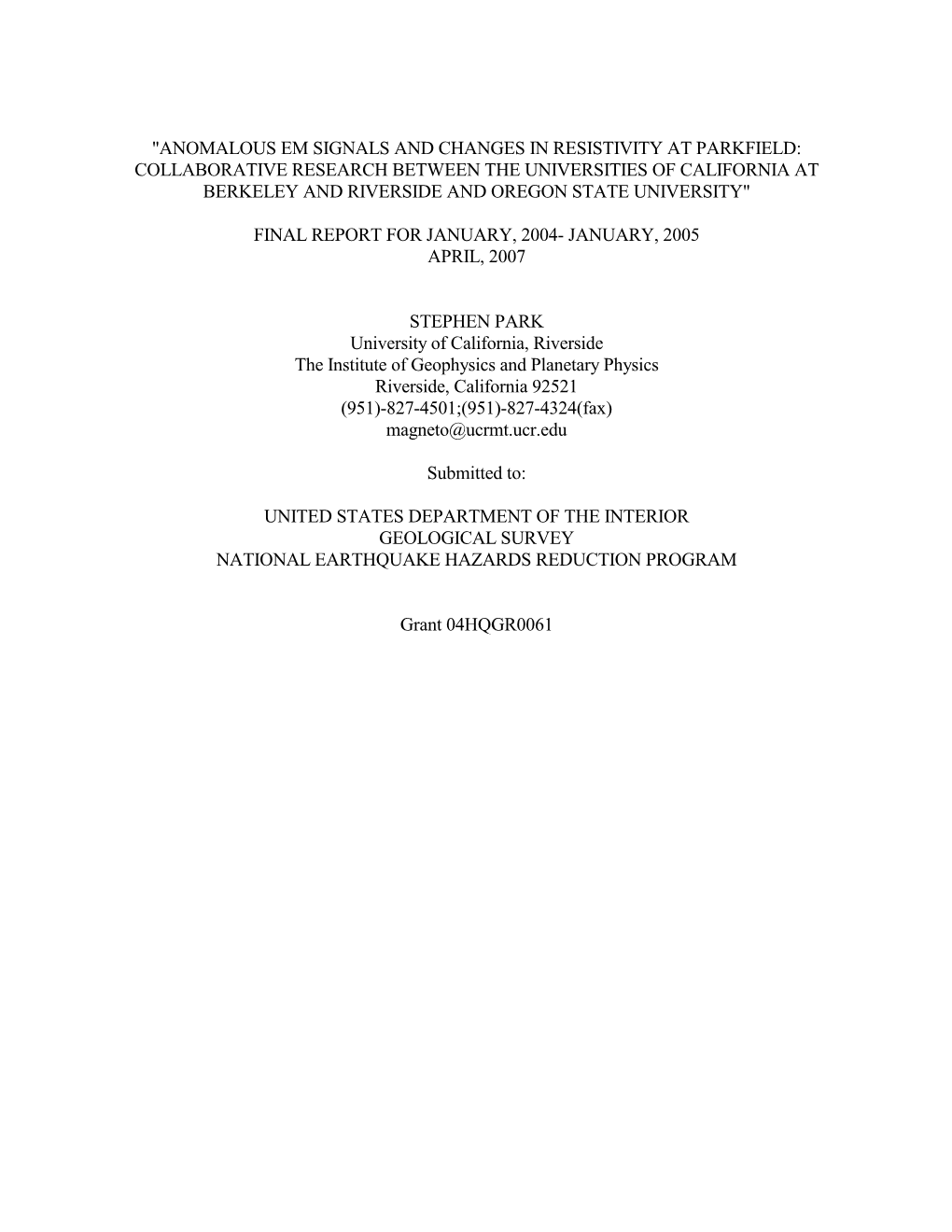
Load more
Recommended publications
-
Application of Artificial Intelligence in Predicting Earthquakes: State-Of-The-Art and Future Challenges
Received September 16, 2020, accepted September 30, 2020. Date of publication xxxx 00, 0000, date of current version xxxx 00, 0000. Digital Object Identifier 10.1109/ACCESS.2020.3029859 Application of Artificial Intelligence in Predicting Earthquakes: State-of-the-Art and Future Challenges MD. HASAN AL BANNA1, (Associate Member, IEEE), KAZI ABU TAHER1, M. SHAMIM KAISER 2, (Senior Member, IEEE), MUFTI MAHMUD 3, (Senior Member, IEEE), MD. SAZZADUR RAHMAN2, (Member, IEEE), A. S. M. SANWAR HOSEN 4, (Member, IEEE), AND GI HWAN CHO 4, (Member, IEEE) 1Department of Information and Communication Technology, Bangladesh University of Professionals, Dhaka 1216, Bangladesh 2Institute of Information Technology, Jahangirnagar University, Dhaka 1342, Bangladesh 3Department of Computer Science, Nottingham Trent University, Nottingham NG11 8NS, U.K. 4Division of Computer Science and Engineering, Jeonbuk National University, Jeonju 54896, South Korea Corresponding authors: Mufti Mahmud ([email protected]; [email protected]) and Gi Hwan Cho ([email protected]) This work was supported in part by fellowship number 19FS12048 from the Information and Communication Technology Division of the Government of the People's Republic of Bangladesh. ABSTRACT Predicting the time, location and magnitude of an earthquake is a challenging job as an earthquake does not show specific patterns resulting in inaccurate predictions. Techniques based on Artificial Intelligence (AI) are well known for their capability to find hidden patterns in data. In the case of earthquake prediction, these models also produce a promising outcome. This work systematically explores the contributions made to date in earthquake prediction using AI-based techniques. A total of 84 scientific research papers, which reported the use of AI-based techniques in earthquake prediction, have been selected from different academic databases. -
Article Count
Nat. Hazards Earth Syst. Sci., 21, 1785–1806, 2021 https://doi.org/10.5194/nhess-21-1785-2021 © Author(s) 2021. This work is distributed under the Creative Commons Attribution 4.0 License. Long-term magnetic anomalies and their possible relationship to the latest greater Chilean earthquakes in the context of the seismo-electromagnetic theory Enrique Guillermo Cordaro1,2, Patricio Venegas-Aravena1,3,4, and David Laroze5 1Observatorios de Radiación Cósmica y Geomagnetismo, Departamento de Física, FCFM, Universidad de Chile, Casilla 487-3, Santiago, Chile 2Facultad de Ingeniería, Universidad Autónoma de Chile, Pedro de Valdivia 425, Santiago, Chile 3Department of Structural and Geotechnical Engineering, School of Engineering, Pontificia Universidad Católica de Chile, Vicuña Mackenna 4860, Macul, Santiago, Chile 4Research Center for Integrated Disaster Risk Management (CIGIDEN), Santiago, Chile 5Instituto de Alta Investigación, Universidad de Tarapacá, Casilla 7D, Arica, Chile Correspondence: Patricio Venegas-Aravena ([email protected]) Received: 24 October 2020 – Discussion started: 26 November 2020 Revised: 29 April 2021 – Accepted: 10 May 2021 – Published: 11 June 2021 Abstract. Several magnetic measurements and theoretical in order to filter space influence. The FFT method confirms developments from different research groups have shown the rise in the power spectral density in the millihertz range certain relationships with worldwide geological processes. 1 month before each earthquake, which decreases to lower Secular variation in geomagnetic cutoff rigidity, magnetic values some months after earthquake occurrence. The cumu- frequencies, or magnetic anomalies have been linked with lative anomaly method exhibited an increase prior to each spatial properties of active convergent tectonic margins or Chilean earthquake (50–90 d prior to earthquakes) similar to earthquake occurrences during recent years. -
Laboratory Investigation of Coupled Electrical Interaction of Fracturing Rock with Gases Yuji Enomoto1* , Tsuneaki Yamabe1, Shigeki Sugiura2 and Hitoshi Kondo2
Enomoto et al. Earth, Planets and Space (2021) 73:90 https://doi.org/10.1186/s40623-021-01416-1 EXPRESS LETTER Open Access Laboratory investigation of coupled electrical interaction of fracturing rock with gases Yuji Enomoto1* , Tsuneaki Yamabe1, Shigeki Sugiura2 and Hitoshi Kondo2 Abstract In the coupled electric interaction of rock fractures and gas invasion, that is, when gases interact with newly created crack surfaces, the unpaired electrons within the rock crystal defects are thermally stimulated, released into the crack due to the temperature rise at the crack tip via plastic work, and attached to ambient gas molecules to electrify them in a negative state. Using a working hypothesis that this mechanism is the source mechanism of seismo-electromag- netic phenomena, we conducted laboratory experiments in which rocks were fractured with pressurized N 2, CO2, CH4, and hot water vapour. Fractures were induced by a fat-ended indenter equipped with a fow channel, which was loaded against blocks of quartz diorite, gabbro, basalt, and granite. Fracture-induced negatively electrifed gas currents at ~ 25 °C and ~ 160 °C were successfully measured for ~ 100 μs after full development of the crack. The peak electric currents were as high as 0.05–3 μA, depending on the≥ rock species and interaction area of fractured rock and gas and to a lesser extent on the gas species and temperature. The peak current from fracturing granite, which showed higher γ-ray activity, was at least 10 times higher than that from fracturing gabbro, quartz diorite, and basalt. The results supported the validity of the present working hypothesis, that coupled interaction of fracturing rock with deep Earth gases during quasi-static rupture of rocks in the focal zone of a fault might play an important role in the generation of pre- and co-seismic electromagnetic phenomena. -
Experimental Study on the Propagation of Seismic Electromagnetic Signals (SEMS) Using a Mini- Geographic Model of the Taiwan Strait
289 by Qinghua Huang1 and Motoji Ikeya2 Experimental study on the propagation of seismic electromagnetic signals (SEMS) using a mini- geographic model of the Taiwan Strait 1 International Frontier Program on Earthquake Research, The Institute of Physical and Chemical Research (RIKEN), at Earthquake Prediction Research Center, Tokai University, 3-20-1, Orido, Shimizu 424-8610, Japan; E-mail: [email protected] 2 Department of Earth and Space Sciences, Graduate School of Science, Osaka University, 1-1 Machikaneyama, Toyonaka, Osaka 560-0043, Japan. Propagation patterns of seismic electromagnetic signals mechanism of EM signals associated with faulting (Huang and (SEMS) of the 1994 M 7.3 Taiwan Strait earthquake Ikeya, 1998b). It would be necessary to investigate experimentally the propa- have been simulated experimentally using a geographic gation of SEMS, till a scientifically credible theory has been made to model of the Taiwan-Fujian region. The power intensity explain those reported long distance propagation. Motivated by the above a granite slab for electromagnetic (EM) waves waveguide concept, a scale-model experiment on the propagation of EM waves in several waveguides has been developed (Ikeya et al., transmitted from the source antenna in the slab was 1997a; Huang and Ikeya, 1998a). Application to two earthquakes in mapped. In this model, aluminum foil was used to simu- Greece indicated some aspect of long distance propagation of SES late the ocean and the lower ionosphere and the under- (Varotsos et al., 1996) may possibly be related to the distribution of sea and land (Huang and Ikeya, 1998a). ground conductive layer were simulated by aluminum In the present experiment, propagation of EM waves from the plates. -
Earthquake-Related Electromagnetic Study in China
Earthquake-related Electromagnetic Study in China Qinghua Huang1, Hengxin Ren2, Xiaofei Chen2, Peng Han3, Yufeng Lin1, Zhanhui Li1, Dunzhu Li1 1 Peking University 2 University of Science and Technology of China 3 Chiba University Contents z Earthquake prediction in China z Earthquake-related electromagnetics in China z Future study on earthquake-related electromagnetics Law of the People’s Republic of China on Protecting Against and Mitigating Earthquake Disasters Article 16. The State adopts the practice of unified release of earthquake prediction. Short-term and imminent earthquake prediction shall be released by the people's governments of provinces, autonomous regions and municipalities directly under the Central Government in accordance with the procedures prescribed by the State 1998 Council … . Conrtesy of Qifu Chen and Kelin Wang Main precursors monitored by China Earthquake Administration (CEA) Seismology Deformation Electromagnetism Underground fluid Distribution of Earthquakes in China (1900 — 2004) M ≥ 5.0 Precursor monitoring network in China 地磁 Geomagnetic 140 地电 Geoelectric 80 重力 Gravity 15 形变 Crustal Deformation 140 应力 Stress-strain 54 水化 Hydrogeochemistry 90 水位 Under Ground Water 240 Deaths: 8064 1328 ~240,000 41 >80,000 Cultural Revolution Conrtesy of Qifu Chen and Kelin Wang Haicheng Tangshan Xingtai Conrtesy of Qifu Chen and Kelin Wang Prediction of the 1975 Haicheng earthquake (Ms 7.3) An unusually pronounced M 7.3 Haicheng earthquake foreshock sequence triggered imminent prediction. Massive amateur participation had educational effects but made no contribution to prediction. Conrtesy of Qifu Chen and Kelin Wang Tangshan (Ms 7.8), 1976, expected seismic intensity VI. No seismic design was required. Actual intensity in earthquake: XI. -
Seismic Electric Signals (SES) and Earthquakes: a Review of an Updated VAN Method and Competing Hypotheses for SES Generation and Earthquake Triggering
Author's Pre-print. This is a non-final version of an article published in final form in Helman, D. S. (2020). Seismic electric signals (SES) and earthquakes: A review of an updated VAN method and competing hypotheses for SES generation and earthquake triggering. Physics of the Earth and Planetary Interiors. In press. Published article: https://doi.org/10.1016/j.pepi.2020.106484 Published under CC BY 4.0 Non-Commercial No Derivatives License Seismic electric signals (SES) and earthquakes: A review of an updated VAN method and competing hypotheses for SES generation and earthquake triggering Daniel S. Helman, Ph.D. Education Division, College of Micronesia-FSM, Yap, Federated States of Micronesia [email protected] - [email protected] Abstract Electromagnetic phenomena are sometimes associated with seismic events, but earthquake prediction using seismic electric signals (SES) has not been seriously considered since the early 1990s. There are several causes: (1) false alarms that have created panics in Greece, and (2) a strong critique of the Varotsos- Alexopoulos-Nomicos (VAN) method used there. An updated VAN method that includes time series analysis has made successful medium-range predictions, and short-range predictions when coupled with seismic time series analysis. Four types of potential mechanisms to create precursory SES relying on deformation effects, temperature effects, ore-mineral movement or groundwater variation are reviewed. Data relevant to each are insufficient to favor a single mechanism. Records of the number of false positive and missed events for an updated VAN method have not been well maintained. False positives appear to be abundant and render the method unusable. -
Correlation Between Abnormal Trends in the Spontaneous Fields of Tectonic
Earthq Sci DOI 10.1007/s11589-017-0180-9 RESEARCH PAPER Correlation between abnormal trends in the spontaneous fields of tectonic plates and strong seismicities Da-Cheng Tan . Jian-Cun Xin Received: 18 January 2017 / Accepted: 6 April 2017 Ó The Author(s) 2017. This article is an open access publication Abstract Tectonic activities, electrical structures, and analysis (Geller 1996; Wyss et al. 1997; Uyeda 2000). electromagnetic environments are major factors that affect Complex electromagnetic environments, dynamically rich the stability of spontaneous fields. The method of corre- observation data, developing observation techniques and lating regional synchronization contrasts (CRSC) can theoretical understanding, and basic dysfunctional short- determine the reliability of multi-site data trends or short- impending predictions of catastrophic earthquakes cause impending anomalies. From 2008 to 2013, there were three credibility problems in geoelectric field observations and strong earthquake cluster periods in the North–South short-impending predictions (Geller 1996; Huang 2006). seismic belt that lasted for 8–12 months. By applying the If the confirmation of the presence of background vari- CRSC method to analyze the spontaneous field ESP at 25 ation trends in the geoelectric fields of the site is possible sites of the region in the past 6 years, it was discovered that before the occurrence of catastrophic earthquakes, then the for each strong earthquake cluster period, the ESP strength implementation of corresponding short-impending geo- of credible anomalous trends was present at minimum 30% electric field information analysis will have a more credible of the stations. In the southern section of the Tan-Lu fault basis. -
Rethinking Earthquake-Related DC-ULF Electromagnetic Phenomena: Towards a Physics-Based Approach
Nat. Hazards Earth Syst. Sci., 11, 2941–2949, 2011 www.nat-hazards-earth-syst-sci.net/11/2941/2011/ Natural Hazards doi:10.5194/nhess-11-2941-2011 and Earth © Author(s) 2011. CC Attribution 3.0 License. System Sciences Rethinking earthquake-related DC-ULF electromagnetic phenomena: towards a physics-based approach Q. Huang1,2 1Department of Geophysics, School of Earth and Space Sciences, Peking University, Beijing 100871, China 2The Key Laboratory of Orogenic Belts and Crustal Evolution, MOE, Beijing 100871, China Received: 15 November 2010 – Revised: 13 June 2011 – Accepted: 28 September 2011 – Published: 4 November 2011 Abstract. Numerous electromagnetic changes possibly re- changes before the 1976 Tangshan earthquake (Zhao and lated with earthquakes have been independently reported and Qian, 1978, 1994). After the early report of geoelectric po- have even been attempted to apply to short-term prediction tential changes before the 1969 Ms = 7.4 Bohai earthquake in of earthquakes. However, there are active debates on the China (Central Earthquake Working Committee, 1969) and above issue because the seismogenic process is rather com- the Kamchatka earthquakes in Russia (Sobolev, 1975), three plicated and the studies have been mainly empirical (i.e. a physicists in Greece (Varotsos, Alexopoulos, and Nomicos, kind of experience-based approach). Thus, a physics-based abbreviated as VAN hereafter) started to make a series ob- study would be helpful for understanding earthquake-related servation test of geoelectric potential changes and developed electromagnetic phenomena and strengthening their applica- the so-called VAN method, which is a short-term earthquake tions. As a potential physics-based approach, I present an in- prediction method using the Seismic Electric Signals (SES) tegrated research scheme, taking into account the interaction extracted from the continuous observing data of geoelectric among observation, methodology, and physical model. -
Prediction Earthquakes
Prediction Of Earthquakes Euan Macpherson Fraser Submitted for the Degree of M.Sc. at The University of Glasgow in the Department of Mathematics, October 1997. ProQuest Number: 13834233 All rights reserved INFORMATION TO ALL USERS The quality of this reproduction is dependent upon the quality of the copy submitted. In the unlikely event that the author did not send a com plete manuscript and there are missing pages, these will be noted. Also, if material had to be removed, a note will indicate the deletion. uest ProQuest 13834233 Published by ProQuest LLC(2019). Copyright of the Dissertation is held by the Author. All rights reserved. This work is protected against unauthorized copying under Title 17, United States C ode Microform Edition © ProQuest LLC. ProQuest LLC. 789 East Eisenhower Parkway P.O. Box 1346 Ann Arbor, Ml 48106- 1346 GLASGOW u n iversity library ;i L iios'3 V eiASGOWUHWERSIff ($RM Abstract We start by introducing the reader to the mechanisms behind earthquake production, including the Elastic Rebound Theory and the theory of Plate Tectonics. We also discuss the quantification of earthquakes in terms of magnitude, intensity and energy released. A short review of the history of earthquake prediction is also given, along with a discussion of some of the latest attempts at discovering seismic precursors. The VAN method, and the controversy surrounding it are discussed in some depth in chapter 5, while the theory of Seismic Gaps is introduced in chapter 6. Experiments using cellular automata to model earthquake production are performed in chapter 7, and the resulting analysis presented. -
Electromagnetic Pre-Earthquake Precursors
ence Sci & C h li rt m a a E t i f c Petraki et al., J Earth Sci Clim Change 2015, 6:1 Journal of o C l h a a DOI: 10.4172/2157-7617.1000250 n n r g u e o J Earth Science & Climatic Change ISSN: 2157-7617 Review Article OpenOpen Access Access Electromagnetic Pre-earthquake Precursors: Mechanisms, Data and Models-A Review Petraki E1, Nikolopoulos D2*, Nomicos C3, Stonham J1, Cantzos D4, Yannakopoulos P2 and Kottou S5 1Brunel University, Department of Engineering and Design, Kingston Lane, Uxbridge, Middlesex UB8 3PH, London, UK 2TEI of Piraeus, Department of Electronic Computer Systems Engineering, Petrou Ralli and Thivon 250, GR-12244 Aigaleo, Athens, Greece 3TEI of Athens, Department of Electronic Engineering, Agiou Spyridonos, GR-12243, Aigaleo, Athens, Greece 4TEI of Piraeus, Department of Automation Engineering, Petrou Ralli and Thivon 250, GR-12244 Aigaleo, Greece 5University of Athens, Medical School, Department of Medical Physics, Mikras Asias 75, GR-11527 Goudi, Athens, Greece Abstract This paper is a survey of pre-earthquake short-term electromagnetic precursors. Extensive studies were carried out the last decades in analysis of electromagnetic emissions from 10-3 Hz up to MHz. These signals were analyzed through visual, statistical and chaotic techniques. The paper presents cumulative elements from scientific investigations performed during the last 40 years in this area. Physical models have also been developed for the interpretation of production and propagation of electromagnetic radiation during the fracture process. The Ultra Low Frequency (ULF) fracture-re-lated emissions attempted to be explained via magneto-hydrodynamic, piezomagnetic and electroki-netic effects whereas for the kHz-MHz observations the model of asperities was proposed. -
Preface to the Special Issue on Electromagnetic Phenomena Related to Seismic and Volcanic Activities from EMSEV in 2016
Earthq Sci (2017) 30(4):165–166 https://doi.org/10.1007/s11589-017-0194-3 PREFACE Preface to the special issue on electromagnetic phenomena related to seismic and volcanic activities from EMSEV in 2016 Xuemin Zhang . Katsumi Hattori . Valerio Tramutoli Published online: 29 November 2017 Ó The Author(s) 2017. This article is an open access publication EMSEV, Electromagnetic Studies of Earthquake and experts from 12 countries attended this conference, and 100 Volcanoes, set up in 2002, is a joint academic organization papers have been presented. A lot of new achievements and by multi-associations in IUGG (International Union of substantial discussions have been accomplished. Geodesy and Geophysics), including IAGA (International With the development of space detection technologies Association of Geomagnetism and Aeronomy), IASPEI since last century in the world, seismo-electromagnetic (International Association of Seismology and Physics of monitoring has been gradually constructed to be a stereo the Earth’s Interior), IAVCEI (International Association of observing system. The new results in EMSEV 2016 illus- Volcanology and Chemistry of the Earth’s Interior). On trated that, not only the traditional ground-based observa- August 25–29, 2016, the 2016 International EMSEV tions such as electric field, magnetic field, apparent Workshop on the observation and understanding of the resistivity, electromagnetic emissions, have been devel- various kinds of electromagnetic phenomena associated oped quickly, but also the seismo-ionospheric detection with earthquakes and volcanic eruptions was held in attracted more and more attention including ionosonde, Lanzhou, Gansu Province. It is the first EMSEV meeting VLF radio wave, Schumann resonance, and satellite elec- held in Chinese mainland. -
A Report from the RIKEN International Frontier Research Project on Earthquakes (IFREQ)
<Back to Index> TAO, Vol. 15, No. 3, 269-310, September 2004 A Report from the RIKEN International Frontier Research Project on Earthquakes (IFREQ) Seiya Uyeda1,*, Toshiyasu Nagao1 and Haruo Tanaka1 (Manuscript received 6 April 2004, in final form 11 June 2004) ABSTRACT Mitigation of seismic hazards requires integration of science and hu- man action, namely the science of earthquakes, anti-seismic engineering and socio-political measures. In this paper, we will be primarily examining the problem of saving human life from earthquakes. Loss of human life from earthquakes is caused overwhelmingly by the collapse of houses and buildings within less than a few minutes of the main shocks. When struc- tural damage is reduced, most other seismic hazards will also be reduced. On top of that, if short-term prediction could be made, casualties would be further reduced dramatically. We review the state of the art in short-term prediction, in particular recent progress in approaches using electromag- netic phenomena. The RIKEN International Frontier Research Project on Earthquakes (IFREQ) has demonstrated the existence of pre-seismic ultra-low frequency (ULF) geo-electric potential changes, a claim that has long been made by the Greek VAN group. In addition, IFREQ has been able to verify the precursory signatures of ULF geomagnetic variations. A review is also made for another promising method using pre-seismic over-the-horizon trans- mission of VHF (~100MHz) FM radio waves. Thus, the reinforcement of existing structures and enhancement of short-term prediction research are the two keys for seismic hazard mitigation. (Key words: Loss of life, Short-term earthquake prediction, Seismo-electromagnetic phenomena) 1 Earthquake Prediction Research Center, Tokai University, Shizuoka, Japan * Corresponding author address: Prof.