Voluminous Eruption from a Zoned Magma Body After an Increase in Supply Rate at Axial Seamount
Total Page:16
File Type:pdf, Size:1020Kb
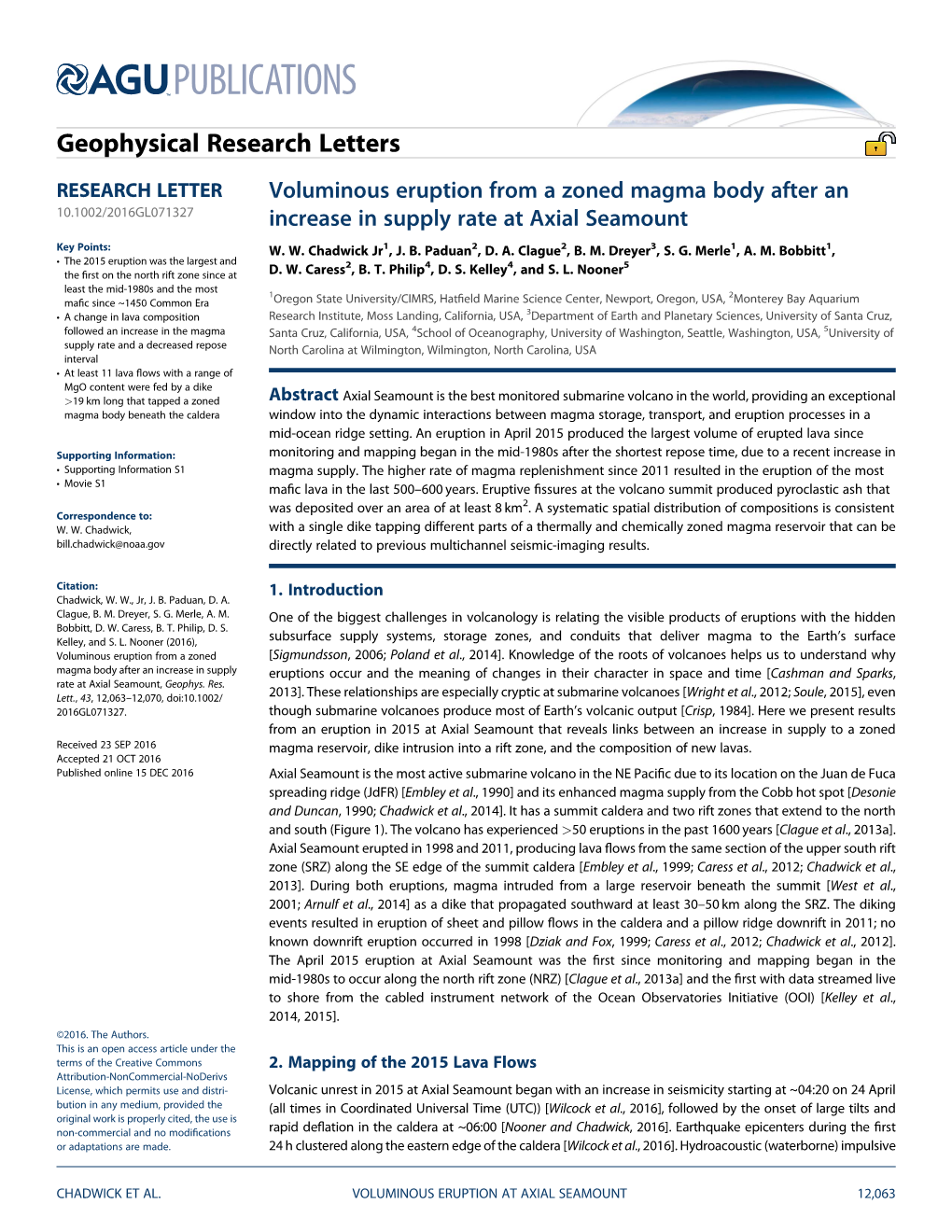
Load more
Recommended publications
-
Geology, Geochemistry and Earthquake History of Loieihi Seamount, Hawaiei's Youngest Volcano
ARTICLE IN PRESS Chemie der Erde ] (]]]]) ]]]–]]] www.elsevier.de/chemer INVITED REVIEW Geology, geochemistry and earthquake history of Lo¯"ihi Seamount, Hawai"i’s youngest volcano Michael O. Garciaa,Ã, Jackie Caplan-Auerbachb, Eric H. De Carloc, M.D. Kurzd, N. Beckera aDepartment of Geology and Geophysics, University of Hawai"i, Honolulu, HI 96822, USA bAlaska Volcano Observatory, U.S.G.S., Alaska Science Center, Anchorage, AK 99508, USA cDepartment of Oceanography, University of Hawai"i, Honolulu, HI 96822, USA dDepartment of Marine Chemistry and Geochemistry, Woods Hole Oceanographic Institution, Woods Hole, MA 02543, USA Received 6 June 2005; accepted 20 September 2005 Abstract A half-century of investigations are summarized here on the youngest Hawaiian volcano, Lo¯"ihi Seamount. It was discovered in 1952 following an earthquake swarm. Surveying in 1954 determined it has an elongate shape, which is the meaning of its Hawaiian name. Lo¯"ihi was mostly forgotten until two earthquake swarms in the 1970s led to a dredging expedition in 1978, which recovered young lavas. The recovery of young lavas motivated numerous expeditions to investigate the geology, geophysics, and geochemistry of this active volcano. Geophysical monitoring, including a real- time submarine observatory that continuously monitored Lo¯"ihi’s seismic activity for 3 months, captured some of the volcano’s earthquake swarms. The 1996 swarm, the largest recorded in Hawai"i, was preceded earlier in the year by at least one eruption and accompanied by the formation of a 300-m deep pit crater, Pele’s Pit. Seismic and petrologic data indicate that magma was stored in a 8–9 km deep reservoir prior to the 1996 eruption. -
Posteruption Enhancement of Hydrothermal Activity: a 33-Year, Multieruption Time Series at Axial Seamount (Juan De Fuca Ridge)
RESEARCH ARTICLE Posteruption Enhancement of Hydrothermal Activity: A 10.1029/2018GC007802 33‐Year, Multieruption Time Series at Axial Key Points: • Water column surveys, 1985‐2017, Seamount (Juan de Fuca Ridge) show that hydrothermal plume rise Edward T. Baker1,2 , Sharon L. Walker2 , William W. Chadwick Jr3 , height and turbidity identify the last 1,2 1,2 1,2 three Axial Seamount eruptions David A. Butterfield , Nathaniel J. Buck , and Joseph A. Resing • Posteruptive enhancement of 1 2 hydrothermal activity lasted 2‐5 Joint Institution for the Study of the Atmosphere and Ocean, University of Washington, Seattle, WA, USA, NOAA/ years posteruption, totaling Pacific Marine Environmental Laboratory, Seattle, WA, USA, 3NOAA Pacific Marine Environmental Laboratory, ~10 years over the survey duration Newport, OR, USA • Posteruption heat flux increased sixfold, implying that fluxes based on noneruptive activity alone will ‐ underestimate the long‐term flux Abstract Mid ocean ridge eruptions, initiating or revitalizing hydrothermal discharge and disrupting seafloor ecosystems, occur regularly as a consequence of plate spreading. Evaluating their impact on Supporting Information: long‐term hydrothermal discharge requires information on the scale and duration of any posteruption • Supporting Information S1 enhancement. Here we describe a unique hydrothermal plume time series of annual (or more frequent) • Figure S1 fi • Data Set S1 observations at Axial Seamount vent elds from 1985 through 2017, missing only 7 years. Axial, a hot spot • Data Set S2 volcano astride the Juan de Fuca Ridge, experienced eruptions in 1998, 2011, and 2015. In 1998 and 2011 • Data Set S3 lava flooded the SE caldera and south rift zone, but in 2015 most lava was extruded in a series of flows • Data Set S4 extending ~20 km down the north rift zone. -
Cenozoic Changes in Pacific Absolute Plate Motion A
CENOZOIC CHANGES IN PACIFIC ABSOLUTE PLATE MOTION A THESIS SUBMITTED TO THE GRADUATE DIVISION OF THE UNIVERSITY OF HAWAI`I IN PARTIAL FULFILLMENT OF THE REQUIREMENTS FOR THE DEGREE OF MASTER OF SCIENCE IN GEOLOGY AND GEOPHYSICS DECEMBER 2003 By Nile Akel Kevis Sterling Thesis Committee: Paul Wessel, Chairperson Loren Kroenke Fred Duennebier We certify that we have read this thesis and that, in our opinion, it is satisfactory in scope and quality as a thesis for the degree of Master of Science in Geology and Geophysics. THESIS COMMITTEE Chairperson ii Abstract Using the polygonal finite rotation method (PFRM) in conjunction with the hotspot- ting technique, a model of Pacific absolute plate motion (APM) from 65 Ma to the present has been created. This model is based primarily on the Hawaiian-Emperor and Louisville hotspot trails but also incorporates the Cobb, Bowie, Kodiak, Foundation, Caroline, Mar- quesas and Pitcairn hotspot trails. Using this model, distinct changes in Pacific APM have been identified at 48, 27, 23, 18, 12 and 6 Ma. These changes are reflected as kinks in the linear trends of Pacific hotspot trails. The sense of motion and timing of a number of circum-Pacific tectonic events appear to be correlated with these changes in Pacific APM. With the model and discussion presented here it is suggested that Pacific hotpots are fixed with respect to one another and with respect to the mantle. If they are moving as some paleomagnetic results suggest, they must be moving coherently in response to large-scale mantle flow. iii List of Tables 4.1 Initial hotspot locations . -
Seismic Precursors and Magma Ascent Before the April 2011 Eruption at Axial Seamount
LETTERS PUBLISHED ONLINE: 10 JUNE 2012 | DOI: 10.1038/NGEO1490 Seismic precursors and magma ascent before the April 2011 eruption at Axial Seamount R. P. Dziak1*, J. H. Haxel1, D. R. Bohnenstiehl2, W. W. Chadwick Jr1, S. L. Nooner3, M. J. Fowler1, H. Matsumoto1 and D. A. Butterfield4 Volcanoes at spreading centres on land often exhibit seismicity seismicity associated with the opening of a hydraulic fracture. As and ground inflation months to years before an eruption, magma leaves the reservoir, the overlying ground surface typically caused by a gradual influx of magma to the source reservoir1–4. subsides. The timing of this deflation signal often coincides with Deflation and seismicity can occur on time scales of hours to seismic activity, but there also are examples where the earth- days, and result from the injection of magma into adjacent quake swarms are delayed by several hours relative to the onset rift zones5–8. Volcanoes at submarine rift zones, such as of deflation2,5,6. Similarly, surface eruptions have been reported Axial Seamount in the northeast Pacific Ocean, have exhibited contemporaneously or up to several hours after deflation began. In similar behaviour9–12, but a direct link between seismicity, other cases, dykes do not break the surface and eruptions are absent seafloor deformation and magma intrusion has never been altogether. In comparison, only two other submarine-rift-zone demonstrated. Here we present recordings from ocean-bottom eruptions have been observed in situ, one with seismic and temper- hydrophones and an established array of bottom-pressure ature sensors (2005–2006 East Pacific Rise eruption sequence) and recorders that reveal patterns of both microearthquakes and the other with geodetic sensors (1998 Axial Seamount; refs 10–12). -
Microbiology of Seamounts Is Still in Its Infancy
or collective redistirbution of any portion of this article by photocopy machine, reposting, or other means is permitted only with the approval of The approval portionthe ofwith any articlepermitted only photocopy by is of machine, reposting, this means or collective or other redistirbution This article has This been published in MOUNTAINS IN THE SEA Oceanography MICROBIOLOGY journal of The 23, Number 1, a quarterly , Volume OF SEAMOUNTS Common Patterns Observed in Community Structure O ceanography ceanography S BY DAVID EmERSON AND CRAIG L. MOYER ociety. © 2010 by The 2010 by O ceanography ceanography O ceanography ceanography ABSTRACT. Much interest has been generated by the discoveries of biodiversity InTRODUCTION S ociety. ociety. associated with seamounts. The volcanically active portion of these undersea Microbial life is remarkable for its resil- A mountains hosts a remarkably diverse range of unusual microbial habitats, from ience to extremes of temperature, pH, article for use and research. this copy in teaching to granted ll rights reserved. is Permission S ociety. ociety. black smokers rich in sulfur to cooler, diffuse, iron-rich hydrothermal vents. As and pressure, as well its ability to persist S such, seamounts potentially represent hotspots of microbial diversity, yet our and thrive using an amazing number or Th e [email protected] to: correspondence all end understanding of the microbiology of seamounts is still in its infancy. Here, we of organic or inorganic food sources. discuss recent work on the detection of seamount microbial communities and the Nowhere are these traits more evident observation that specific community groups may be indicative of specific geochemical than in the deep ocean. -
Sr Isotopic Variations Along the Juan De Fuca Ridge
University of South Florida Scholar Commons Marine Science Faculty Publications College of Marine Science 9-1984 Sr Isotopic Variations along the Juan de Fuca Ridge Jacqueline Eaby U.S. Geological Survey, [email protected] David A. Clague U.S. Geological Survey John R. Delaney University of Washington Follow this and additional works at: https://scholarcommons.usf.edu/msc_facpub Part of the Life Sciences Commons Scholar Commons Citation Eaby, Jacqueline; Clague, David A.; and Delaney, John R., "Sr Isotopic Variations along the Juan de Fuca Ridge" (1984). Marine Science Faculty Publications. 1321. https://scholarcommons.usf.edu/msc_facpub/1321 This Article is brought to you for free and open access by the College of Marine Science at Scholar Commons. It has been accepted for inclusion in Marine Science Faculty Publications by an authorized administrator of Scholar Commons. For more information, please contact [email protected]. JOURNAL OF GEOPHYSICAL RESEARCH, VOL. 89, NO. B9, PAGES 7883-7890, SEPTEMBER 10, 1984 Sr IsotopicVariations Along the Juande Fuca Ridge JACQUELINEEABY AND DAVID A. CLAGUE U.S. GeologicalSurvey, Menlo Park, California JOHN R. DELANEY OceanographyDepartment, University of Washington,Seattle Srisotopic ratios of 39glass and microcrystalline basalt samples along the Juan de Fuca Ridge and 1 glasssample from BrownBear Seamount are at the lowerend of the rangefor normalmid-oceanic ridge basalt(MORB)' the average87Sr/S6Sr ratio is 0.70249+ 0.00014(2-a). Althoughsubtle variations exist alongstrike of the ridge,the Sr isotopedata do not showsystematic variation relative to the proposed CobbHotspot. The isotopicdata are inconsistentwith an enrichedmantle-plume origin for the Cobb- EickelbergSeamount chain, as has beenproposed for Iceland,the Azores,and the Galapagosspreading center.Sr isotopicratios of samplescollected north and southof the Cobb offsetare identical,although minor elementratios indicate that theseregions have chemicallydistinct mantle sources. -
Axial Seamount
AXIAL SEAMOUNT - WIRED AND RESTLESS: A Cabled Submarine Network Enables Real-time, Tracking of a Mid-Ocean Ridge Eruption and Live Video of an Active Hydrothermal System Juan de Fuca Ridge, NE Pacific John R. Delaney1, Deborah S. Kelley1, Aaron Marburg2, Kim Juniper3 and Friedrich Knuth4 Mark Stoermer1, and Hunter Hadaway1, University of Victoria3 Ocean Networks Canada,Victoria School of Oceanography1 and Applied Physics B.C., Canada; 4 Rutgers University, Department of Marine Laboratory2 University of Washington and Coastal Sciences, New Brunswick, Seattle, WA, 98195 New Jersey Abstract—The most scientifically diverse and technologically understood owing to their remote locations beneath one to four advanced component of the National Science Foundations’ kilometers of seawater. As a consequence, submarine volcano- $386M investment in the Ocean Observatories Initiative (OOI), hydrothermal systems have never been observed-monitored involves 900 kilometers of high power and bandwidth electro- continuously. Nor have their immediate effects on overlying optical cable extending from Pacific City, OR, across active marine ecosystems been assessed because they are most active portions of the Juan de Fuca tectonic plate and up into the just before, during, and shortly after unpredictable eruptions overlying ocean. Completed on time and under budget in when the systems are the most energetic. Tracking the long- October, 2014, this mesoscale fiber-optic sensor array enables term behavior of even a small section of this powerful real-time, high-bandwidth, 2-way communication with seafloor planetary-scale submarine MOR could contribute substantially and water-column sensor networks across: 1) a portion of the to our understanding of a host of complex oceanic processes global Mid-Ocean Ridge (MOR), 2) a section of the Cascadia Subduction Zone, and, 3) a cross-section of the California triggered by numerous eruptions along this global submarine Current, a component of the North Pacific Gyre. -
The Axial Seamount: Life on a Vent
The Axial Seamount: Life on a Vent Timeframe Description 50 minutes This activity asks students to understand, and build a food web to Target Audience describe the interdependent relationships of hydrothermal vent organisms. Hydrothermal vents were only discovered in 1977, and as Grades 5th- 8th more vents are explored we are finding out more about the unique creatures that live there. In Life on a Vent students will learn about Suggested Materials vent organisms, their feeding relationships, and use that information • Hydrothermal vent organism cards to construct a food web. • Poster paper Objectives • Marking pens Students will: • Sticky notes • Make a food web diagram of the hydrothermal vent community • Painter's tape (to show connections) • Show the flow of energy and materials in a vent ecosystem • Learn about organisms that live in extreme environments and use chemosynthesis to produce energy • Make claims and arguments about each organisms place in the food web Essential Questions What do producers and consumers use as energy at hydrothermal vent ecosystems, and how does that energy travel through the trophic levels of the ecosystem? Background Information Hydrothermal Vents, How do They Form? Under sea volcanoes at spreading ridges and convergent plate boundaries produce underwater geysers, known as hydrothermal vents. They form as seawater seeps deep into the ocean's crust. As the seawater seeps deeper into the Earth, it interacts with latent heat from nearby magma chambers, which are possibly fueling a nearby volcano. Once the freezing cold water heats up deep near the Contact: crust, it begins to rise. As the extremely hot seawater rises, it melts SMILE Program the rocks it passes by leaching chemicals and metals from them [email protected] through high heat chemical reactions. -
Explosive Processes During the 2015 Eruption of Axial Seamount, As Recorded by Seafloor Hydrophones
Western Washington University Western CEDAR Geology Faculty Publications Geology 4-2017 Explosive Processes during the 2015 Eruption of Axial Seamount, as Recorded by Seafloor Hydrophones Jacqueline Caplan-Auerbach Western Washington University, [email protected] R. P. Dziak NOAA/Pacific aM rine Environmental Laborartory J. Haxel Oregon State University D. R. Bohnenstiehl North Carolina State University at Raleigh C. Garcia University of Washington Follow this and additional works at: https://cedar.wwu.edu/geology_facpubs Part of the Geology Commons, and the Volcanology Commons Recommended Citation Caplan-Auerbach, J., R. P. Dziak, J. Haxel, D. R. Bohnenstiehl, and C. Garcia (2017), Explosive processes during the 2015 eruption of Axial Seamount, as recorded by seafloor yh drophones, Geochem. Geophys. Geosyst., 18, 1761–1774, doi:10.1002/2016GC006734. This Article is brought to you for free and open access by the Geology at Western CEDAR. It has been accepted for inclusion in Geology Faculty Publications by an authorized administrator of Western CEDAR. For more information, please contact [email protected]. PUBLICATIONS Geochemistry, Geophysics, Geosystems RESEARCH ARTICLE Explosive processes during the 2015 eruption of Axial 10.1002/2016GC006734 Seamount, as recorded by seafloor hydrophones Key Points: J. Caplan-Auerbach1, R. P. Dziak2, J. Haxel2,3, D. R. Bohnenstiehl4, and C. Garcia5 A full eruption cycle at Axial seamount was captured by a network 1Department of Geology, Western Washington University, Bellingham, Washington, -
Oceanographyra Spocietyhy
OceThe OfficiaaL MaganZineog of the Oceanographyra Spocietyhy CITATION Rubin, K.H., S.A. Soule, W.W. Chadwick Jr., D.J. Fornari, D.A. Clague, R.W. Embley, E.T. Baker, M.R. Perfit, D.W. Caress, and R.P. Dziak. 2012. Volcanic eruptions in the deep sea. Oceanography 25(1):142–157, http://dx.doi.org/10.5670/oceanog.2012.12. DOI http://dx.doi.org/10.5670/oceanog.2012.12 COPYRIGHT This article has been published inOceanography , Volume 25, Number 1, a quarterly journal of The Oceanography Society. Copyright 2012 by The Oceanography Society. All rights reserved. USAGE Permission is granted to copy this article for use in teaching and research. Republication, systematic reproduction, or collective redistribution of any portion of this article by photocopy machine, reposting, or other means is permitted only with the approval of The Oceanography Society. Send all correspondence to: [email protected] or The Oceanography Society, PO Box 1931, Rockville, MD 20849-1931, USA. downLoaded from http://www.tos.org/oceanography OCEANIC SPREADING CENTER PROCESSES | Ridge 2000 PROGRAM RESEARCH Volcanic Eruptions in the Deep Sea BY KEnnETH H. RUBIN, S. ADAM SOULE, WILLIAM W. CHADWICK JR., DANIEL J. FORNARI, DAVID A. CLAGUE, RobERT W. EMBLEY, EDWARD T. BAKER, MICHAEL R. PERFIT, DAVID W. CAREss, AND RobERT P. DZIAK Eruption of molten lapilli, ash, and sulfur-rich fumes at Hades vent, West Mata Volcano. 142 Oceanography | Vol. 25, No. 1 AbsTRACT. Volcanic eruptions are important events in Earth’s cycle of magma eruptions, we also discuss submarine generation and crustal construction. Over durations of hours to years, eruptions eruptions in other settings because produce new deposits of lava and/or fragmentary ejecta, transfer heat and magmatic volcanism is a continuum of conditions volatiles from Earth’s interior to the overlying air or seawater, and significantly and processes across the full range of modify the landscape and perturb local ecosystems. -
Revised Magmatic Source Models for the 2015 Eruption at Axial Seamount Including Estimates of Fault‐Induced Deformation
RESEARCH ARTICLE Revised Magmatic Source Models for the 2015 Eruption 10.1029/2020JB019356 at Axial Seamount Including Estimates Key Points: ‐ • Fault motion during the 2015 of Fault Induced Deformation eruption of Axial Seamount William L. Hefner1 , Scott L. Nooner1 , William W. Chadwick Jr2 , contributed up to several 3 centimeters of vertical motion to the and DelWayne R. Bohnenstiehl deformation field 1 2 • Including estimates of fault‐induced Department of Earth and Ocean Sciences, University of North Carolina Wilmington, Wilmington, NC, USA, Hatfield uplift results in a revised Marine Science Center, Oregon State University/Cooperative Institute for Marine Resources Studies, Newport, OR, USA, deformation source 2.1 km to the 3Department of Marine, Earth, and Atmospheric Sciences and Center for Geospatial Analytics, North Carolina State WSW from the previously modeled University, Raleigh, NC, USA source • Future source models should include fault motion to provide better estimates of active magma Abstract Axial Seamount is an active submarine volcano located at the intersection of the Cobb hot spot movement through time at Axial and the Juan de Fuca Ridge (45°57′N, 130°01′W). Bottom pressure recorders captured co‐eruption Seamount subsidence of 2.4–3.2 m in 1998, 2011, and 2015, and campaign‐style pressure surveys every 1–2 years have provided a long‐term time series of inter‐eruption re‐inflation. The 2015 eruption occurred shortly after the Supporting Information: • Supporting Information S1 Ocean Observatories Initiative (OOI) Cabled Array came online providing real‐time seismic and deformation observations for the first time. Nooner and Chadwick (2016, https://doi.org/10.1126/science. -
Seafloor Magnetotelluric Sounding Above Axial Seamount
GEOPHYSICAL RESEARCH LETTERS, VOL. 23, NO. 17, PAGES 2275-2278, AUGUST 15, 1996 SeafloorMagnetotelluric Sounding Above Axial Seamount Graham Heinson Schoolof Earth Sciences,Flinders University, Bedford Park, Australia Steven Constable Instituteof Geophysicsand Planetary Physics, University of CaliforniaSan Diego, La Jolla Antony White Schoolof Earth Sciences,Flinders University, Bedford Park, Australia Abstract. Axial Seamountis a large,active, ridge axis volcano Instrumentation and Data locatedon the centralsegment of the Juande FucaRidge in Three setsof magnetotelluric(MT) instrumentswere de- the northeastPacific Ocean. Magnetotelluric (MT) datahave ployedfrom 3 ra July to the7 th September, 1994. The GPS- beencollected at threesites, approximately 4 km apartaround determineddeployment locations and depths(Plate 1) were the easternrim of the volcano,during a 65-day deployment. MT responses,in the bandwidth of 102- 105s, arealmost for Pele 45ø 55.47' N, 129ø 59.03' W (1550 m), Macques45 ø 57.48' N, 129ø 58.98' W (1520 m) andUlysses 45 ø 59.50' N, isotropic,with a weakly-definedprincipal direction of strike 129ø 59.81' W (1490 m). parallelto the maintopographic trends of Axial Seamount, Electric fields were recordedby the two-componentshort- and are relativelyflat over the wholebandwidth. Apparent resistivitiesare of the orderof 7 - 20 •m, andphases are as armed electrometerdescribed in Constableand Cox [1996], with the high-gainAC amplifiersreplaced by lower gain DC low as 30ø at the shortperiods. Diagonalterms of the MT amplifiers.The least-countwas 0.006/•V andthe samplerate tensorare an orderof magnitudesmaller than the off-diagonal 1 s. The magnetometers,developed at Flinders University terms,suggesting that three-dimensional effects on the data [White, 1979], areinstalled in standard17 inchglass instrument areminimal.