A Dissertation Submitted to the Temple University Graduate Board
Total Page:16
File Type:pdf, Size:1020Kb
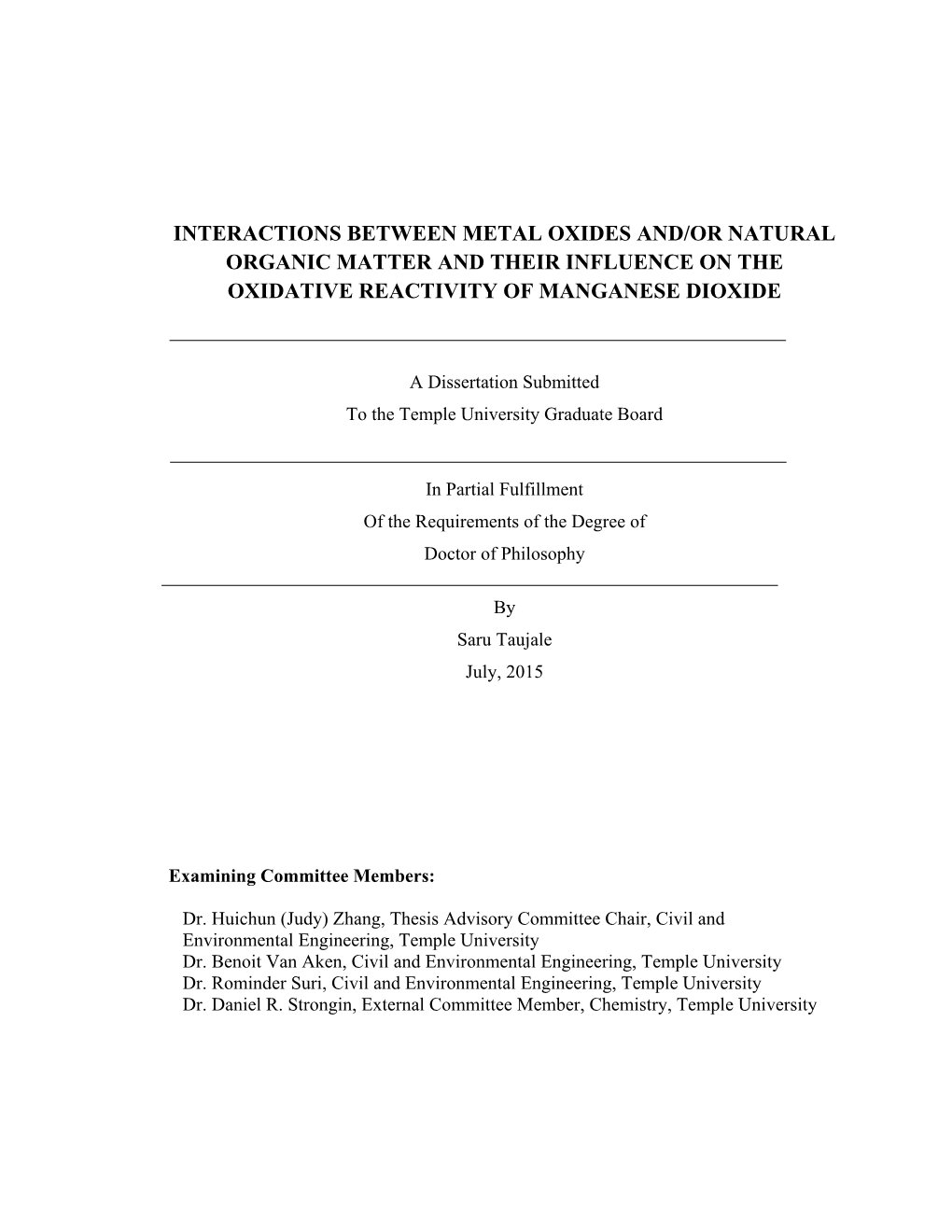
Load more
Recommended publications
-
Mineral Formation and Sorption Mechanisms in Marine Ferromanganese-Rich Sediments
Mineral Formation and Sorption Mechanisms In Marine Ferromanganese-rich Sediments Amy Leanne Atkins Submitted in accordance with the requirements for the degree of Doctor of Philosophy The University of Leeds School of Earth and Environment October 2014 The candidate confirms that the work submitted is her own, except where work, which has formed part of jointly authored publications has been included. The contributions of the candidate and the other authors to this work have been indicated overleaf. The candidate confirms that appropriate credit has been given where reference has been made to the work of others. This copy has been supplied on the understanding that it is copyright material and that no quotation from this thesis may be published without proper acknowledgement. ii Declaration Chapter 4 is a reproduction of a peer-reviewed publication in the journal Geochimica et Cosmochimica Acta as: Atkins A. L., Shaw S., Peacock C. L. (2014) Nucleation and growth of todorokite: Implications for trace-metal cycling in marine sediments. Geochim. Cosmochim. Acta. 144, 109-125. TEM images presented in chapter 4 were collected by Michael Ward at the Leeds Electron Microscopy and Spectroscopy centre with the guidance of the candidate. All other experimental work has been conducted by the candidate. The interpretation of the experimental data, and preparation of the manuscript has been undertaken by the candidate with the help and guidance of her supervisors: Caroline L. Peacock and Sam Shaw. Chapter 5 is a reproduction of a manuscript currently in the final stages of preparation for submission to the journal Geochimica et Cosmochimica Acta as: Atkins A. -
Crystallographic Determination of Wild Type, Mutant and Substrate
Copyright is owned by the Author of the thesis. Permission is given for a copy to be downloaded by an individual for the purpose of research and private study only. The thesis may not be reproduced elsewhere without the permission of the Author. Crystallographic Determination of Wild Type, Mutant and SubstrateSubstrate---- Analogue Inhibited Structures of Bacterial Members of a Family of Superoxide Dismutases bybyby Simon Hardie Oakley Submitted as part of the requirements for the degree of Doctor of Philosophy Institute of Fundamental Sciences, Chemistry Massey University, New Zealand 2009 i "Part of the inhumanity of the computer is that, once it is competently programmed and working smoothly, it is completely honest." Isaac Asimov (1920-92), author, inventor of the word “robotics”. ii 0.1 Abstract Crystallographic Determination of Wild Type, Mutant and Substrate-Analogue Inhibited Structures of Bacterial Members of a Family of Superoxide Dismutases The iron and manganese superoxide dismutases are a family of metallo-enzymes with highly conserved protein folds, active sites and dimer interfaces. They catalyse the elimination of the cytotoxic free radical superoxide to molecular oxygen and hydrogen peroxide by alternate reduction then oxidation of the active- site with the concomitant transfer of protons from the solvent. There are many key aspects of enzymatic function that lack a structural explanation. The focus of this study is on three crystal structures. The iron-substituted manganese superoxide dismutase from Escherichia coli complexed with azide, a substrate-mimicking inhibitor, was solved to 2.2 Å. This “wrong” metal form shows a binding pattern seen previously in the manganese superoxide dismutase from Thermus thermophilus. -
Surface Geochemistry of the Clay Minerals
Proc. Natl. Acad. Sci. USA Vol. 96, pp. 3358–3364, March 1999 Colloquium Paper This paper was presented at the National Academy of Sciences colloquium ‘‘Geology, Mineralogy, and Human Welfare,’’ held November 8–9, 1998 at the Arnold and Mabel Beckman Center in Irvine, CA. Surface geochemistry of the clay minerals GARRISON SPOSITO*†,NEAL T. SKIPPER‡,REBECCA SUTTON*, SUNG-HO PARK*, ALAN K. SOPER§, AND JEFFERY A. GREATHOUSE¶ *Earth Sciences Division, Mail Stop 90y1116, Ernest Orlando Lawrence Berkeley National Laboratory, University of California, Berkeley, CA 94720; ‡Department of Physics and Astronomy, University College, Gower Street, London WC1E 6BT, United Kingdom; §ISIS Facility, Rutherford Appleton Laboratory, Chilton, Didcot, Oxfordshire OX11 0QX, United Kingdom; and ¶Department of Chemistry, University of the Incarnate Word, 4301 Broadway, San Antonio, TX 78209 ABSTRACT Clay minerals are layer type aluminosilicates (6) and in the construction of environmental liners (7). Major that figure in terrestrial biogeochemical cycles, in the buff- impact on industrial organic synthesis comes in the many ering capacity of the oceans, and in the containment of toxic designed catalysts developed from clay minerals with adsorbed waste materials. They are also used as lubricants in petroleum polymeric cations (8). extraction and as industrial catalysts for the synthesis of many Certain clay minerals are isostructural with mica (1, 2) but organic compounds. These applications derive fundamentally are not as well crystallized because of random isomorphic from the colloidal size and permanent structural charge of cation substitutions in their structure (9, 10). These cation clay mineral particles, which endow them with significant substitutions lead to a negative net surface charge that induces surface reactivity. -
Arsenic Adsorption Onto Minerals: Connecting Experimental Observations with Density Functional Theory Calculations
Minerals 2014, 4, 208-240; doi:10.3390/min4020208 OPEN ACCESS minerals ISSN 2075-163X www.mdpi.com/journal/minerals Article Arsenic Adsorption onto Minerals: Connecting Experimental Observations with Density Functional Theory Calculations Heath D. Watts 1,*, Lorena Tribe 2 and James D. Kubicki 1,3,* 1 Department of Geosciences, The Pennsylvania State University, University Park, PA 16802, USA 2 Division of Science, The Pennsylvania State University, Berks, Reading, PA 19610, USA; E-Mail: [email protected] 3 Earth and Environmental Systems Institute, The Pennsylvania State University, University Park, PA 16802, USA * Authors to whom correspondence should be addressed; E-Mails: [email protected] (H.D.W.); [email protected] (J.D.K.); Tel.: +1-814-865-3951 (J.D.K.); Fax: +1-814-867-2378 (J.D.K). Received: 24 December 2013; in revised form: 25 February 2014 / Accepted: 6 March 2014 / Published: 27 March 2014 Abstract: A review of the literature about calculating the adsorption properties of arsenic onto mineral models using density functional theory (DFT) is presented. Furthermore, this work presents DFT results that show the effect of model charge, hydration, oxidation state, and DFT method on the structures and adsorption energies for AsIII and AsV onto Fe3+-(oxyhydr)oxide cluster models. Calculated interatomic distances from periodic planewave and cluster-model DFT are compared with experimental data for AsIII and AsV adsorbed to Fe3+-(oxyhydr)oxide models. In addition, reaction rates for the adsorption of AsV on α-FeOOH (goethite) (010) and Fe3+ (oxyhydr)oxide cluster models were calculated using planewave and cluster-model DFT methods. Keywords: arsenic; density functional theory (DFT); kinetics; thermodynamics; adsorption; computational chemistry; planewave DFT; reaction rates; As—Fe bond distances Minerals 2014, 4 209 1. -
Kinetics and Mechanisms of Electron Transfer Reactions of Metal Complexes Ormond Jerry Parker Iowa State University
Iowa State University Capstones, Theses and Retrospective Theses and Dissertations Dissertations 1968 Kinetics and mechanisms of electron transfer reactions of metal complexes Ormond Jerry Parker Iowa State University Follow this and additional works at: https://lib.dr.iastate.edu/rtd Part of the Physical Chemistry Commons Recommended Citation Parker, Ormond Jerry, "Kinetics and mechanisms of electron transfer reactions of metal complexes " (1968). Retrospective Theses and Dissertations. 4619. https://lib.dr.iastate.edu/rtd/4619 This Dissertation is brought to you for free and open access by the Iowa State University Capstones, Theses and Dissertations at Iowa State University Digital Repository. It has been accepted for inclusion in Retrospective Theses and Dissertations by an authorized administrator of Iowa State University Digital Repository. For more information, please contact [email protected]. This dissertation has been microfihned exactly as received 69-9882 PARKER, Ormond Jerry, 1939- KINETICS AND MECHANISMS OF ELECTRON TRANSFER REACTIONS OF METAL COMPLEXES. Iowa State University, Ph.D., 1968 Chemistry, physical University Microfilms. Inc., Ann Arbor, Michigan KINETICS AND MECHANISMS OF ELECTRON TRANSFER REACTIONS OF METAL COMPLEXES by Ormond Jerry Parker A Dissertation Submitted to the Graduate Faculty in Partial Fulfillment of The Requirements for the Degree of DOCTOR OF PHILOSOPHY Major Subject: Physical Chemistry Approved: Signature was redacted for privacy. IffyCharge of Maj<6r Work Signature was redacted for privacy. -
Adsorption of Selenite on Heat Treated Sea Nodule Residue
International Journal of Advanced Chemical Science and Applications (IJACSA) ________________________________________________________________________ Adsorption of Selenite on Heat Treated Sea Nodule Residue 1P.K.Satapathy, 2T.R.Mohanta, 3A.K.Sahoo 1P.G. Department of Chemistry, North Orissa University, Baripada 2Department of Chemistry, F.M. Junior College, Balasore Email: [email protected] Adsorption of selenite/selenate on hydrous oxides of ABSTRACT: - The present study was carried out to investigate the adsorption characteristics of sea nodule iron, manganese, and aluminum [2, 3-6] appears to be an efficient and inexpensive method for removing trace residue (SNR) heated at 400°C towards aqueous selenite. contaminants. Selenite adsorption on manganese nodule Physico-chemical characterization revealed that the leached residue was a complex mixture of oxides of mainly surface was also studied by Parida et al[7]. However adsorption of selenite has not yet been studied using heat manganese and iron along with MnCO3. Adsorption studies were carried out at varying the pH, selenite ion treated sea nodule residue. So the present study is an concentration, SNR dosage and in presence of competitive attempt to use 400°C heated sea nodule residue sample ions. It was found that the selenite adsorption increased as adsorbent towards adsorption of selenium. with pH reaches maximum at pH 5.0 and then decreased. Further it was found that the percentage of selenite II. EXPERIMENTAL adsorption increased with increasing amount of adsorbent Materials (SNR) and decreased with increasing initial concentration of selenite. It was observed that the percentage of Leached residue sample (SNR), generated after adsorption of selenite decreased with increasing reduction roast-ammoniacal leaching process were concentration of externally added ions. -
Surface Chemistry of Al and Si (Hydr)Oxides, with Emphasis On
i Surface chemistry of Al and Si (hydr)oxides, with emphasis on nano-sized gibbsite (α-Al(OH)3) by Jörgen Rosenqvist Department of Chemistry, Inorganic Chemistry Umeå University Umeå, Sweden ii ISBN 91-7305-245-0 Printed by Solfjädern Offset AB Umeå, Sweden, 2002 iii Surface chemistry of Al and Si (hydr)oxides, with emphasis on nano-sized gibbsite (α-Al(OH)3) by Jörgen Rosenqvist Akademisk avhandling som med tillstånd av rektorsämbetet vid Umeå Universitet för erhållande av Teknologie Doktorsexamen framlägges till offentlig granskning vid kemiska institutionen, sal KB3B1, fredagen den 31 maj 2002, kl. 13.00. Fakultetsopponent: Dr. Gerhard Furrer, Institute of Terrestrial Ecology, ETH Zürich, Schweiz. iv Title: Surface chemistry of Al and Si (hydr)oxides, with emphasis on nano-sized gibbsite (α-Al(OH)3). Author: Jörgen Rosenqvist Address: Department of Chemistry, Inorganic Chemistry, Umeå University, SE-901 87 Umeå, Sweden Abstract: This thesis contains an introduction to the surface chemistry of minerals in aqueous environment, and a summary of five manuscripts concerning adsorption reactions at the surfaces of nano-sized gibbsite (α-Al(OH)3), amorphous silica and kaolinite. Nano-sized gibbsite was synthesized and thoroughly characterized using X-ray diffraction, high-resolution transmission electron microscopy, atomic force microscopy and Fourier transform infrared spectroscopy. The adsorption of protons and the development of charge at the surfaces were studied using high precision potentiometry and zeta potential measurements. The results showed that singly coordinated surface sites at the particle edges protonate/deprotonate, while ion pairs with the medium ions are formed at doubly coordinated surface sites at the basal planes. -
EXAFS Study of Sr Sorption to Illite, Goethite, Chlorite, and Mixed Sediment Under Hyperalkaline Conditions Adam J
Article pubs.acs.org/Langmuir EXAFS Study of Sr sorption to Illite, Goethite, Chlorite, and Mixed Sediment under Hyperalkaline Conditions Adam J. Fuller,†,∥ Samuel Shaw,‡ Caroline L. Peacock,† Divyesh Trivedi,§ and Ian T. Burke*,† † School of Earth and Environment, University of Leeds, Leeds LS2 9JT, United Kingdom ‡ School of Earth, Atmospheric and Environmental Science, University of Manchester, Manchester M13 9PL, United Kingdom § National Nuclear Laboratory Ltd, Chadwick House, Warrington Road, Birchwood Park, Warrington WA3 6AE, United Kingdom *S Supporting Information ABSTRACT: Strontium is an important contaminant radionuclide at many former nuclear sites. This paper investigates the effect of changing pH and ionic strength on the sorption of Sr to a range of common soil minerals. Specifically it focuses on the sorption of Sr onto illite, chlorite, goethite, and a mixed sediment. The interplay between ionic strength and pH was determined by varying the background ionic strength of the system using both NaCl (for a constant pH) and NaOH (to also vary pH). Under conditions of moderate pH, Sr sorption decreased with increasing ionic strength, due to competition between the Na and Sr atoms for the outer-sphere complexes. However, where increasing ionic strength was accompanied by increasing pH, Sr sorption remained high. This suggested that Sr was sorbed to the minerals without competition from background Na ions. Extended X-ray absorption fine structure (EXAFS) spectra confirmed that at highly alkaline pH (>12.5) Sr was forming inner-sphere complexes on the surfaces of all minerals. This specific adsorption of the Sr (as SrOH+) explains why it was still adsorbed to the minerals under very high ionic strength conditions and was not out- competed by Na. -
Density Functional Theory Calculations on the Sorption Of
Density Functional Theory Calculations on the Sorption of Organoarsenicals onto Hydrated Iron (III) Oxyhydroxide Clusters by Adrian Adamescu A thesis presented to the University of Waterloo in fulfillment of the thesis requirement for the degree of Doctor of Philosophy in Chemistry Waterloo, Ontario, Canada, 2017 © Adrian Adamescu 2017 Examining Committee Membership The following served on the Examining Committee for this thesis. The decision of the Examining Committee is by majority vote. External Examiner NAME: Dr. Randall Dumont Title: Associate Professor Supervisor(s) NAME: Dr. Marcel Nooijen Title: Associate Professor NAME: Dr. Ian Hamilton Title: Professor NAME: Dr. Hind Al-Abadleh Title: Associate Professor Internal Member NAME: Dr. Pierre-Nicholas Roy Title: Professor Internal-external Member NAME: Dr. Philippe Van Cappellen Title: Canada Excellence Research Chair Other Member(s) NAME: Dr. Michael Denk Title: Associate Professor NAME: Dr. Carol Ptacek Title: Professor ii Author’s Declaration I hereby declare that I am the sole author of this thesis. This is a true copy of the thesis, including any required final revisions, as accepted by my examiners. I understand that my thesis may be made electronically available to the public. iii Abstract Organic forms of arsenic are introduced into the environment by natural and anthropogenic processes and they pose a threat to human health. The fate of these pollutants depends on their interactions with reactive soils components, such as iron oxyhydroxides. Reaction pathway information and transition states are essential for understanding adsorption mechanisms of pollutants at the liquid–solid interface. Density functional theory (DFT) calculations on the energies, optimal geometries, vibrational frequencies for organic arsenical complexes, p-arsanilic acid (pAsA), monomethylarsonic acid (MMA) and dimethylarsinic acid (DMA) with iron oxyhydroxide clusters are performed. -
DOE-ERSP PI MEETING: Abstracts
DOE-ERSP PI MEETING: Abstracts April 3–5, 2006 Warrenton, Virginia Environmental Remediation Sciences Program (ERSP) This work was supported by the Office of Science, Biological and Environmental Research, Environmental Remediation Sciences Division (ERSD), U.S. Department of Energy under Contract No. DE-AC03-76SF00098. Table of Contents Introduction...................................................................................................................... 1 ERSP Program Contacts................................................................................................. 2 Agenda............................................................................................................................ 3 Abstracts........................................................................................................................ 6 Biogeochemistry/Biotransformation...................................................................... 7 Bluhm, Hendrik..................................................................................................... 8 Bolton, Harvey, Jr. ................................................................................................ 9 Coates, John D................................................................................................... 10 Coates, John D................................................................................................... 11 Deng, Baolin....................................................................................................... 12 DePaolo, -
Uranium(VI) Sorption Complexes on Montmorillonite As a Function of Solution Chemistry1
Journal of Colloid and Interface Science 233, 38–49 (2001) doi:10.1006/jcis.2000.7227, available online at http://www.idealibrary.com on Uranium(VI) Sorption Complexes on Montmorillonite as a Function of Solution Chemistry1 , Catherine J. Chisholm-Brause, ,2 John M. Berg,† Robert A. Matzner,‡ and David E. Morris§ 2 School of Marine Science, College of William and Mary, VIMS, Gloucester Point, Virginia 23062; †Nuclear Materials Technology Division and §Chemistry Division, MS J514, Los Alamos National Laboratory, Los Alamos, New Mexico 87545; and ‡United States Environmental Protection Agency, Washington, DC 20460 Received November 16, 1999; revised August 7, 2000 INTRODUCTION We have investigated the effect of changes in solution chem- istry on the nature of uranyl sorption complexes on montmoril- Environmental contaminant releases that contain uranium are lonite (SAz-1) at different surface coverages (1.43–53.6 mol/g). among the more serious problems that must be confronted by Uranyl uptake onto SAz-1 between pH 3 and 7 was determined restoration programs. For example, uranium has been identified in both titration and batch-mode experiments. These pH val- in complex contaminant mixtures at 12 U.S. Department of En- ues result in solutions that contain a range of monomeric and ergy facilities at concentration levels approaching 16,000 ppm oligomeric aqueous uranyl species. Continuous-wave and time- in soils and sediments (1). Uranium mill tailings have also pro- resolved emission spectroscopies were used to investigate the na- duced soil and mine-water contamination plumes having very ture of U(VI) sorbed to SAz-1. A discrete set of uranyl surface high dissolved and solid uranium concentrations at pH values ap- complexes has been identified over a wide range of pH values proaching 2.5 (2, 3). -
Thermodynamic Impact of Mineral Surfaces on Amino Acid Polymerization: Aspartate Dimerization on Two-Line Ferrihydrite, Anatase, and Γ-Alumina
minerals Article Thermodynamic Impact of Mineral Surfaces on Amino Acid Polymerization: Aspartate Dimerization on Two-Line Ferrihydrite, Anatase, and γ-Alumina Norio Kitadai 1,2,*, Kumiko Nishiuchi 2 and Wataru Takahagi 1,3 1 Super-cutting-edge Grand and Advanced Research (SUGAR) Program, Institute for Extra-cutting-edge Science and Technology Avant-garde Research (X-star), Japan Agency for Marine-Earth Science and Technology (JAMSTEC), Yokosuka 237-0061, Japan; [email protected] 2 Earth-Life Science Institute, Tokyo Institute of Technology, Tokyo 152-8550, Japan; [email protected] 3 Department of Chemistry, Graduate School of Science, The University of Tokyo, Tokyo 113-0033, Japan * Correspondence: [email protected] Abstract: The presence of amino acids in diverse extraterrestrial materials has suggested that amino acids are widespread in our solar system, serving as a common class of components for the chemical evolution of life. However, there are a limited number of parameters available for modeling amino acid polymerization at mineral–water interfaces, although the interfacial conditions inevitably exist on astronomical bodies with surface liquid water. Here, we present a set of extended triple-layer model parameters for aspartate (Asp) and aspartyl-aspartate (AspAsp) adsorptions on two-line ferrihydrite, anatase, and γ-alumina determined based on the experimental adsorption data. By combining the parameters with the reported thermodynamic constants for amino acid polymerization in water, we computationally demonstrate how these minerals impact the AspAsp/Asp equilibrium over a wide range of environmental conditions. It was predicted, for example, that two-line ferrihydrite strongly Citation: Kitadai, N.; Nishiuchi, K.; Takahagi, W.