Explosion Temperatures of Metals and Other Elemental Dust Clouds
Total Page:16
File Type:pdf, Size:1020Kb
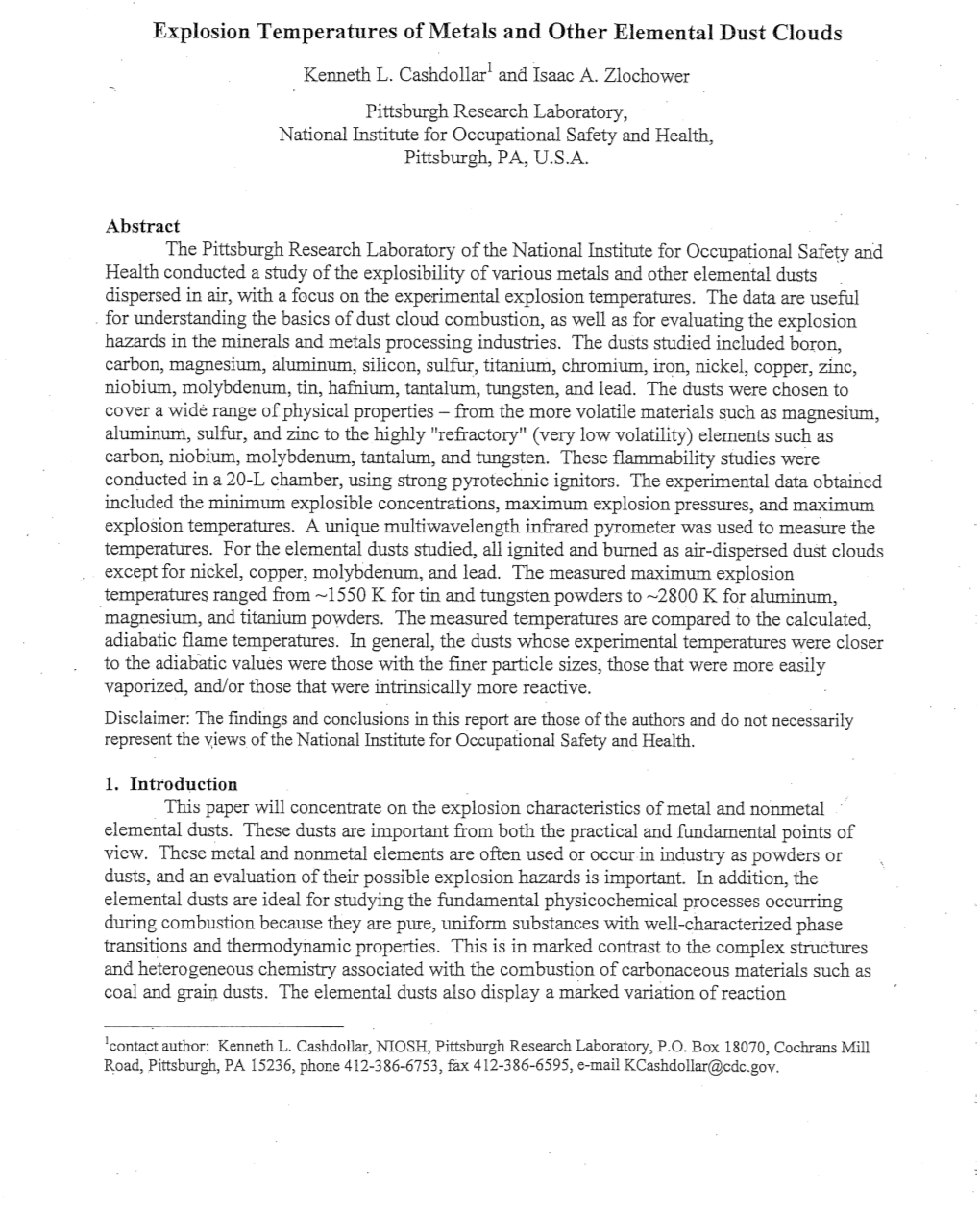
Load more
Recommended publications
-
A Simple Approach to the Supernova Progenitor-Explosion Connection
A Simple Approach to the Supernova Progenitor-Explosion Connection Bernhard Müller Queen's University Belfast Monash Alexander Heger, David Liptai, Joshua Cameron (Monash University) Many potential/indirect observables from core-collapse supernovae, but some of the most direct ones (explosion energies, remnant masses) are heavy elements challenging for SN theory! massive star core-collapse supernovae neutron stars & gravitational waves neutrinos supernova remnants The neutrino-driven mechanism in its modern flavour shock ● Stalled accretion shock still oscillations (“SASI”) pushed outward to ~150km as matter piles up on the PNS, then recedes again convection ● Heating or gain region g develops some tens of ms n i t g a n after bounce e li h o o c ● Convective overturn & shock oscillations “SASI” enhance the efficiency of -heating, shock which finally revives the shock ● Big challenge: Show that this works! Status of 3D Neutrino Hydrodynamics Models with Multi-Group Transport First-principle 3D models: ● Mixed record, some failures ● Some explosions, delayed compared to 2D ● Models close to the threshold So what is missing? 27 M Hanke et al. (2013) 2 3/5 2 −3/5 ⊙ ● Lcrit ∝M˙ M 14 Ma /3 ● → Increase neutrino heating or Reynolds stresses ● Unknown/undetermined microphysics (e.g. Melson et al. 2015)? ● 20 M Melson et al. (2015) Lower explosion threshold in ⊙ SASI-dominated regime (Fernandez 2015)? 15 M⊙ Lentz et al. (2015) ● Better 1D/multi-D progenitor Or with simpler schemes: e.g. IDSA+leakage Takiwaki et al. (2014) models? Challenge: Connecting to Observables Several 50 diagnostic 10 erg with explosion sustained energy accretion . l a ) t 2 e 1 a 0 k 2 ( n Pejcha & Prieto (2015): Explosion energies a J vs. -
Could a Nearby Supernova Explosion Have Caused a Mass Extinction? JOHN ELLIS* and DAVID N
Proc. Natl. Acad. Sci. USA Vol. 92, pp. 235-238, January 1995 Astronomy Could a nearby supernova explosion have caused a mass extinction? JOHN ELLIS* AND DAVID N. SCHRAMMtt *Theoretical Physics Division, European Organization for Nuclear Research, CH-1211, Geneva 23, Switzerland; tDepartment of Astronomy and Astrophysics, University of Chicago, 5640 South Ellis Avenue, Chicago, IL 60637; and *National Aeronautics and Space Administration/Fermilab Astrophysics Center, Fermi National Accelerator Laboratory, Batavia, IL 60510 Contributed by David N. Schramm, September 6, 1994 ABSTRACT We examine the possibility that a nearby the solar constant, supernova explosions, and meteorite or supernova explosion could have caused one or more of the comet impacts that could be due to perturbations of the Oort mass extinctions identified by paleontologists. We discuss the cloud. The first of these has little experimental support. possible rate of such events in the light of the recent suggested Nemesis (4), a conjectured binary companion of the Sun, identification of Geminga as a supernova remnant less than seems to have been excluded as a mechanism for the third,§ 100 parsec (pc) away and the discovery ofa millisecond pulsar although other possibilities such as passage of the solar system about 150 pc away and observations of SN 1987A. The fluxes through the galactic plane may still be tenable. The supernova of y-radiation and charged cosmic rays on the Earth are mechanism (6, 7) has attracted less research interest than some estimated, and their effects on the Earth's ozone layer are of the others, perhaps because there has not been a recent discussed. -
Explosive Chemical Hazards & Risk
Safe Operating Procedure (1/13) EXPLOSIVE CHEMICAL HAZARDS & RISK MINIMIZATION _____________________________________________________________________ (For assistance, please contact EHS at (402) 472-4925, or visit our web site at http://ehs.unl.edu/) Background The Globally Harmonized System (GHS) of classification and labeling of chemicals defines an explosive materials as follows: a solid or liquid substance (or mixture of substances) which is in itself capable by chemical reaction of producing gas at such temperature and pressure and at such speed as to cause damage to the surroundings. Under the GHS system, there are seven divisions for explosives. • Unstable explosives • Division 1.1 – mass explosion hazards (i.e., nearly instant detonation of the entire quantity of explosive present) • Division 1.2 – projection hazards but not mass explosion hazards • Division 1.3 – minor mass explosion or projection hazards • Divisions 1.4 through 1.6 – very insensitive substances; negligible probability of accidental initiation or propagation Explosive chemicals will be identified with the pictogram shown below. In addition, Section 2 of the Safety Data Sheet (SDS) will include one or more of the hazard statements indicated below. • H200 Unstable; explosive • H201 Explosive; mass explosion hazard • H202 Explosive; severe projection hazard • H203 Explosive; fire, blast or projection hazard • H204 Fire or projection hazard • H205 May explode in fire Scope This SOP is limited in scope to those chemicals that meet the GHS definition of an explosive. However, it is important to understand that explosions can occur with chemicals that are not considered GHS explosives. For example, an explosion can occur with large-scale polymerization of a monomer. The reaction of monomers forming polymers is exothermic. -
Blasts from the Past Historic Supernovas
BLASTS from the PAST: Historic Supernovas 185 386 393 1006 1054 1181 1572 1604 1680 RCW 86 G11.2-0.3 G347.3-0.5 SN 1006 Crab Nebula 3C58 Tycho’s SNR Kepler’s SNR Cassiopeia A Historical Observers: Chinese Historical Observers: Chinese Historical Observers: Chinese Historical Observers: Chinese, Japanese, Historical Observers: Chinese, Japanese, Historical Observers: Chinese, Japanese Historical Observers: European, Chinese, Korean Historical Observers: European, Chinese, Korean Historical Observers: European? Arabic, European Arabic, Native American? Likelihood of Identification: Possible Likelihood of Identification: Probable Likelihood of Identification: Possible Likelihood of Identification: Possible Likelihood of Identification: Definite Likelihood of Identification: Definite Likelihood of Identification: Possible Likelihood of Identification: Definite Likelihood of Identification: Definite Distance Estimate: 8,200 light years Distance Estimate: 16,000 light years Distance Estimate: 3,000 light years Distance Estimate: 10,000 light years Distance Estimate: 7,500 light years Distance Estimate: 13,000 light years Distance Estimate: 10,000 light years Distance Estimate: 7,000 light years Distance Estimate: 6,000 light years Type: Core collapse of massive star Type: Core collapse of massive star Type: Core collapse of massive star? Type: Core collapse of massive star Type: Thermonuclear explosion of white dwarf Type: Thermonuclear explosion of white dwarf? Type: Core collapse of massive star Type: Thermonuclear explosion of white dwarf Type: Core collapse of massive star NASA’s ChANdrA X-rAy ObServAtOry historic supernovas chandra x-ray observatory Every 50 years or so, a star in our Since supernovas are relatively rare events in the Milky historic supernovas that occurred in our galaxy. Eight of the trine of the incorruptibility of the stars, and set the stage for observed around 1671 AD. -
The Texas City Disaster
National Hazardous Materials Fusion Center HAZMAT HISTORY The Texas City Disaster The National Hazardous Materials Fusion Center offers Hazmat History as an avenue for responders to learn from the past and apply those lessons learned to future incidents for a more successful outcome. This coincides with the overarching mission of the Fusion Center – to improve hazmat responder safety and enhance the decision‐making process during pre‐planning and mitigation of hazmat incidents. Incident Details: Insert Location and Date Texas City, TX April 16, 1947 Hazardous Material Involved Ammonium Nitrate Fertilizer Type (mode of transportation, fixed facility) Cargo Ship Overview The morning of 16 April 1947 dawned clear and crisp, cooled by a brisk north wind. Just before 8 am, longshoremen removed the hatch covers on Hold 4 of the French Liberty ship Grandcamp as they prepared to load the remainder of a consignment of ammonium nitrate fertilizer. Some 2,086 mt (2,300 t) were already onboard, 798 (880) of which were in the lower part of Hold 4. The remainder of the ship's cargo consisted of large balls of sisal twine, peanuts, drilling equipment, tobacco, cotton, and a few cases of small ammunition. No special safety precautions were in focus at the time. Several longshoremen descended into the hold and waited for the first pallets holding the 45 kg (100 lb) packages to be hoisted from dockside. Soon thereafter, someone smelled smoke, a plume was observed rising between the cargo holds and the ship’s hull, apparently about seven or eight layers of sacks down. Neither a 3.8 L (1 gal) jug of drinking water nor the contents of two fire extinguishers supplied by crew members seemed to do much good. -
Explosives March 2017
Hazard Communication Information Sheet reflecting the US OSHA Implementation of the Globally Harmonized System of Classification and Labelling of Chemicals (GHS) Produced by the SCHC-OSHA Alliance GHS/HazCom Information Sheet Workgroup Info Explosives March 2017 How does OSHA’s Hazard Communication Standard (HCS 2012) define explosives? An explosive chemical is a solid or liquid chemical which is in itself capable by chemical reaction of producing gas at such a temperature and pressure and at such a speed as to cause damage to the surroundings. Pyrotechnic chemicals are included even when they do not evolve gases. A pyrotechnic chemical is a chemical designed to produce an effect by heat, light, sound, gas or smoke or a combination of these as the result of non-detonative self-sustaining exothermic chemical reactions. An explosive item is an item containing one or more explosive chemicals. A pyrotechnic item is an item containing one or more pyrotechnic chemicals. An unstable explosive is an explosive which is thermally unstable and/or too sensitive for normal handling, transport, or use. An intentional explosive is a chemical or item which is manufactured with a view to produce a practical explosive or pyrotechnic effect. How does HCS 2012 classify explosives? The class of explosives includes: a) explosive chemicals; b) explosive items, except devices containing explosive chemicals in such quantity or of such a character that their inadvertent or accidental ignition or initiation does not cause any effect external to the device either by projection, fire, smoke, heat or loud noise; and c) chemicals and items not included in a) and b) above which are manufactured with the view to producing a practical explosive or pyrotechnic effect. -
Chapter 2 EXPLOSIVES
Chapter 2 EXPLOSIVES This chapter classifies commercial blasting compounds according to their explosive class and type. Initiating devices are listed and described as well. Military explosives are treated separately. The ingredi- ents and more significant properties of each explosive are tabulated and briefly discussed. Data are sum- marized from various handbooks, textbooks, and manufacturers’ technical data sheets. THEORY OF EXPLOSIVES In general, an explosive has four basic characteristics: (1) It is a chemical compound or mixture ignited by heat, shock, impact, friction, or a combination of these conditions; (2) Upon ignition, it decom- poses rapidly in a detonation; (3) There is a rapid release of heat and large quantities of high-pressure gases that expand rapidly with sufficient force to overcome confining forces; and (4) The energy released by the detonation of explosives produces four basic effects; (a) rock fragmentation; (b) rock displacement; (c) ground vibration; and (d) air blast. A general theory of explosives is that the detonation of the explosives charge causes a high-velocity shock wave and a tremendous release of gas. The shock wave cracks and crushes the rock near the explosives and creates thousands of cracks in the rock. These cracks are then filled with the expanding gases. The gases continue to fill and expand the cracks until the gas pressure is too weak to expand the cracks any further, or are vented from the rock. The ingredients in explosives manufactured are classified as: Explosive bases. An explosive base is a solid or a liquid which, upon application or heat or shock, breaks down very rapidly into gaseous products, with an accompanying release of heat energy. -
(Usbdc) Explosives Incident Report (Eir) 2016
UNCLASSIFIED UNITED STATES BOMB DATA CENTER (USBDC) EXPLOSIVES INCIDENT REPORT (EIR) 2016 The Annual Explosives Incident Report (EIR) reviews bombing and explosives related incidents and threats from information reported to the United States Bomb Data Center (USBDC) through the Bomb Arson Tracking System (BATS). UNCLASSIFIED UNCLASSIFIED Table of Contents Executive Summary – 2016 ______________________________________________________________________________ 1 Explosion Incidents – 2016 ______________________________________________________________________________ 2 Recoveries – 2016 ________________________________________________________________________________________ 8 Suspicious Packages – 2016 ___________________________________________________________________________ 12 Bomb Threats – 2016 __________________________________________________________________________________ 13 Hoaxes – 2016 __________________________________________________________________________________________ 14 Explosives Thefts/Losses – 2016 _____________________________________________________________________ 16 Contact Information ____________________________________________________________________________________ 19 UNCLASSIFIED UNCLASSIFIED 2016 Explosives Incident Report (EIR) EXECUTIVE SUMMARY Executive Summary – 2016 OPERATING HIGHLIGHTS The 2016 Explosives Incident Report (EIR) is an informational product prepared by the United States Bomb Data Center (USBDC), using incident data reported in the Bomb Arson Tracking System (BATS) by its nearly 2,500 interagency -
Part II EXPLOSIVES INCIDENTS ANALYSIS
If you have issues viewing or .accessing this file contact us at NCJRS.gov. lIP :. I , . •• , .. I , It • ~ • -. I .. • r • -. I , I "$: .; ~.. ,~--.~.~. " I I I I ,.. .~;- ""', ....... ~~-, ~,-- - --------~- .. ". ---------------- Cover: Top Photo: The results of an explosive device that detonated beneath the vehicle as it was traveling through the Kansas City, Missouri, area. The detonation ldlled the driver and severely injured the driver's wife. (Photo .courtesy of Dan Dyer, the News Leader Newspaper.) Bottom Photo: The scene of an explosion in Duncanville, Alabama, that killed the two occupants of the trailer. 12608.1 U.S. Department of Justice National Institute of Justice This document has been reproduced exactly as received from the person or organization originating it. Points of view or opinions stated In this document are those of the authors and do not necessarily represent the official position or policies of the National Institute of Justice. Permission to reproduce this "'IJIi". material has been granted by U.S. Dept. of Treasury/Bureau of Alcohol, Tobacco & Firearms to the National Criminal Justice Reference Service (NCJRS). Further reproduction outside of the NCJRS system requires permis sion of the ~ owner. Dedication This year we have seen a significant increase in the number of injuries sustained by our State and local counterparts in the law enforcement and fire service communities. The dangers inherent to their line of work are a given; however, the ever-changing environment in which they work has increased these dangers. Booby-trapped drug operations and illicit explosives manufac turing operations are but a few of the criminal activities that present such dangers, not only to them but to the public as well. -
Basics of Explosion Protection INTRODUCTION
Basics of Explosion Protection INTRODUCTION HAZARDOUS LOCATIONS Understanding Global Explosion Protection • Class and Division System • Class-Zone System • CENELEC Zone System • IEC INNOVATIVE EXPLOSION PROTECTION by R. STAHL 1-800-782-4357 A INTRODUCTION Basics of Explosion Protection HAZARDOUS LOCATIONS Basics of Explosion Protection HAZARDOUS LOCATIONS Hazardous locations are defined as premises, buildings or parts thereof where fire or explosion hazards may exist due to the presence of flammable gases or vapors, flammable liquids, combustible dusts, or easily ignitable fibers or flyings. Although, flammable gases, vapors and combustible dusts exist almost everywhere, fortunately, they are present only in minute quantities. Simply because flammable gasses or vapors, or combustible dusts are present, there is not necessarily a hazardous location. The quantities or concentrations must be sufficient to present a potential explosion hazard. The electrical codes that deal with these types of hazardous locations areas do not deal with materials such as high explosives, such as dynamite, munitions, or fireworks. Other rules and regulations deal with areas involving these materials. Understanding “Global” Hazardous Location Requirements The evolution of hazardous location electrical codes and standards throughout the world has taken two distinct paths. In North America, a “Class, Division” System has been used for decades as the basis for area classification of hazardous (classified) locations. Because the hazards and methods of protecting electrical equipment against these hazards differ for different materials, hazardous locations are divided into three Classes, and two Divisions. The Classes are based on the type of hazard and the explosive characteristics of the material with the Divisions being based on the occurrence or risk of fire or explosion that the material presents. -
Pure Nuclear Fusion Bomb Propulsion
Pure Nuclear Fusion Bomb Propulsion F.Winterberg University of Nevada, Reno March 2008 1 Abstract Recent progress towards the non-fission ignition of thermonuclear micro-explosions raises the prospect for a revival of the nuclear bomb propulsion idea, both for the fast transport of large payloads within the solar system and the launch into earth orbit without the release of fission products into the atmosphere. To reach this goal three areas of research are of importance: 1) Compact thermonuclear ignition drivers. 2) Fast ignition and deuterium burn. 3) Space-craft architecture involving magnetic insulation and GeV electrostatic potentials. 2 1. Introduction With chemical propulsion manned space flight to the moon is barely possible and only with massive multistage rockets. For manned space flight beyond the moon, nuclear propulsion is indispensible. Nuclear thermal propulsion is really not much better than advanced chemical propulsion. Ion propulsion, using a nuclear reactor driving an electric generator, has a much higher specific impulse, but not enough thrust for short interplanetary transit times, needed for manned missions. This leaves nuclear bomb propulsion as the only credible option. Both its thrust and specific impulse are huge in comparison. For a history of nuclear bomb propulsion, reference is made to a long article by A.R.Martin and A.Bond [1]. Under the name project Orion, it was studied in great detail under the leadership of Theodore Taylor and Freeman Dyson. Its history has been published by George Dyson [2], the son of Freeman Dyson. The project was brought to a sudden halt by the nuclear test ban treaty, motivated by the undesirable release of nuclear fission products into the atmosphere. -
00320773.Pdf
LA-2000 PHYSICS AND MATHEMATICS (TID-4500, 13th ed., Supp,.) LOS ALAMOS SCIENTIFIC LABORATORY OF THE UNIVERSITY OF CALIFORNIA LOS ALAMOS NEW MEXICO REPORT WRITTEN: August 1947 REPORT DISTRIBUTED: March 27, 1956 BLAST WAVE’ Hans A. Bethe Klaus Fuchs Joseph 0. Hirxhfelder John L. Magee Rudolph E. Peierls John van Neumann *This report supersedes LA-1020 and part of LA-1021 =- -l- g$g? ;-E Contract W-7405-ENG. 36 with the U. S. Atomic Energy Commission m 2-0 This report is a compilation of the dedassifid chapters of reports LA.-IO2O and LA-1021, which 11.a.sbeen published becWL’3eof the demnd for the hfcmmatimo with the exception d? CaM@mr 3, which was revised in MN& it reports early work in the field of blast phencmma. Ini$smw’$has the authors had h?ft the b$ Ala.mos kb(mktm’y Wkll this z%po~ was compiled, -they have not had ‘the Oppxrtl.mity to review their [email protected] and make mx.m%xtti.cms to reflect later tiitii~e ., CONTENTS Page Chapter I INTRODUCTION, by Hans A. Bethe 11 1.1 Areas of Discussion 11 1.2 Comparison of Nuclear and Ordinary Explosion 11 1.3 The Sequence of Events in a Blast Wave Produced by a Nuclear Explosion 13 1.4 Radiation 16 1.5 Reflection of Blast Wave, Altitude Effect, etc. 21 1.6 Damage 24 1.7 Measurements of Blast 26 Chapter 2 THE POINT SOURCE SOLUTION, by John von Neumann 27 2.1 Introduction 27 2.2 Analytical Solution of the Problem 30 2.3 Evaluation and Interpretation of the Results 46 Chapter 3 THERMAL RADIATION PHENOMENA, by John L.