I Investigating the Role of VGF and Its Associated Neuropeptides in Post
Total Page:16
File Type:pdf, Size:1020Kb
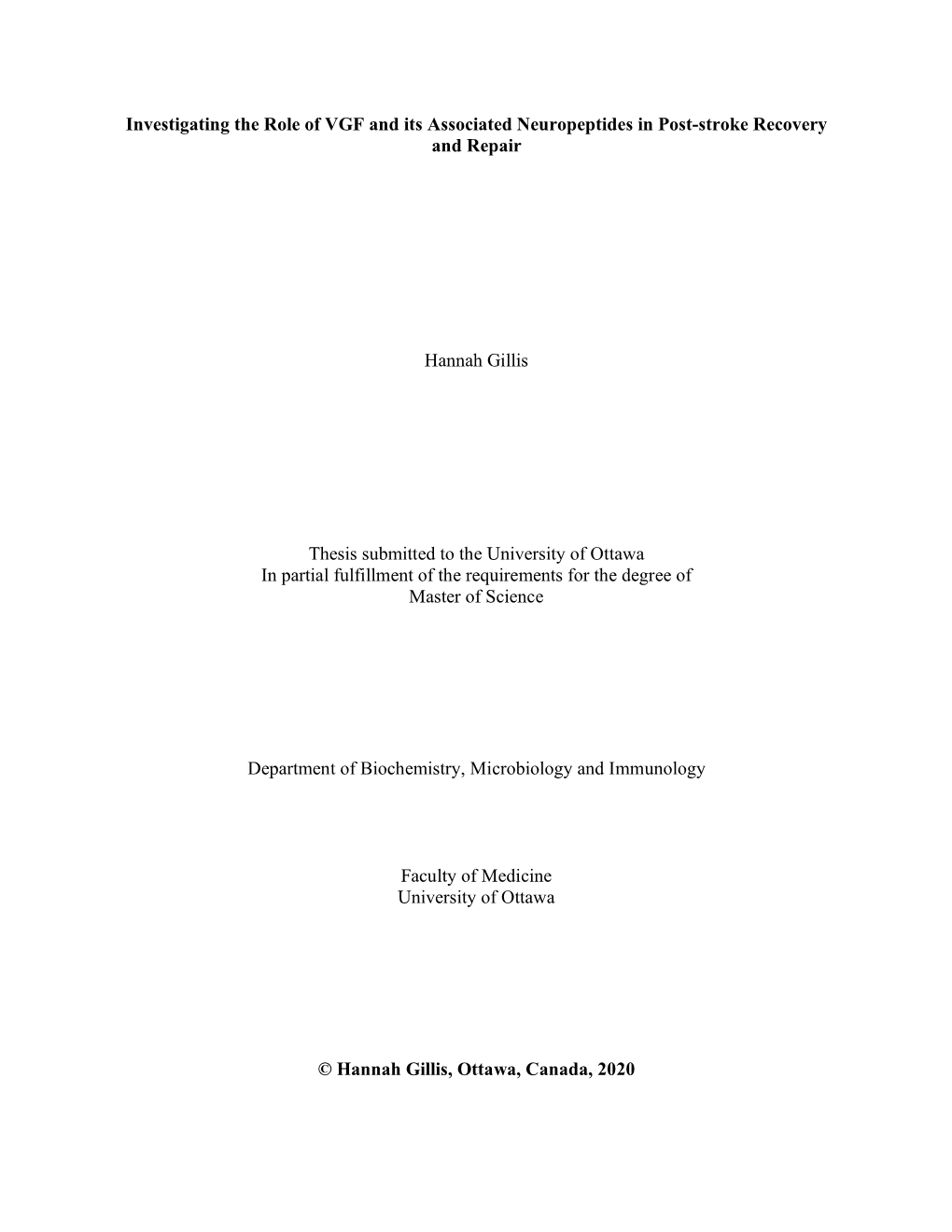
Load more
Recommended publications
-
NS Royal Gazette Part I
Nova Scotia Published by Authority PART 1 VOLUME 217, NO. 13 HALIFAX, NOVA SCOTIA, WEDNESDAY, MARCH 26, 2008 IN THE MATTER OF: The Companies Act, the Nova Scotia Registrar of Joint Stock Companies for Chapter 81, R.S.N.S. 1989, as amended leave to surrender its Certificate of Incorporation. - and - IN THE MATTER OF: The Application of DATED the 25th day of March, 2008. 2126466 Nova Scotia Limited for Leave to Surrender its Certificate of Incorporation Barry D. Horne McInnes Cooper NOTICE 1300-1969 Upper Water Street Purdy’s Wharf Tower II 2126466 NOVA SCOTIA LIMITED hereby gives Halifax NS B3J 3R7 notice pursuant to the provisions of Section 137 of the Solicitor for Custom Industries Canada Corp. Companies Act that it intends to make application to the Nova Scotia Registrar of Joint Stock Companies for 675 March 26-2008 leave to surrender its Certificate of Incorporation. IN THE MATTER OF: The Companies Act, DATED the 18th day of March, 2008. being Chapter 81 of the Revised Statutes of Nova Scotia 1989, as amended R. Daren Baxter - and - McInnes Cooper IN THE MATTER OF: The Application of 1300-1969 Upper Water Street Dimensional Quality Services Company for Purdy’s Wharf Tower II Leave to Surrender its Certificate of Incorporation Halifax NS B3J 3R7 Solicitor for 2126466 Nova Scotia Limited NOTICE 674 March 26-2008 DIMENSIONAL QUALITY SERVICES COMPANY gives notice pursuant to the provisions of Section 137 of IN THE MATTER OF: The Companies Act, the Companies Act (Nova Scotia) that it intends to make Chapter 81, R.S.N.S. -
GSK-3Β in Mouse Fibroblasts Controls Wound
Research article GSK-3β in mouse fibroblasts controls wound healing and fibrosis through an endothelin-1– dependent mechanism Mohit Kapoor,1 Shangxi Liu,1 Xu Shi-wen,2 Kun Huh,1 Matthew McCann,1 Christopher P. Denton,2 James R. Woodgett,3 David J. Abraham,2 and Andrew Leask1 1Division of Oral Biology and Department of Physiology and Pharmacology, University of Western Ontario, London, Ontario, Canada. 2Centre for Rheumatology, University College London, London, United Kingdom. 3Samuel Lunenfeld Research Institute, Mount Sinai Hospital, Toronto, Ontario, Canada. Glycogen synthase kinase–3 (GSK-3) is a widely expressed and highly conserved serine/threonine protein kinase encoded by 2 genes, GSK3A and GSK3B. GSK-3 is thought to be involved in tissue repair and fibrogen- esis, but its role in these processes is currently unknown. To investigate the function of GSK-3β in fibroblasts, we generated mice harboring a fibroblast-specific deletion of Gsk3b and evaluated their wound-healing and fibrogenic responses. We have shown that Gsk3b-conditional-KO mice (Gsk3b-CKO mice) exhibited accelerated wound closure, increased fibrogenesis, and excessive scarring compared with control mice. In addition, Gsk3b- CKO mice showed elevated collagen production, decreased cell apoptosis, elevated levels of profibrotic α-SMA, and increased myofibroblast formation during wound healing. In cultured Gsk3b-CKO fibroblasts, adhesion, spreading, migration, and contraction were enhanced. Both Gsk3b-CKO mice and fibroblasts showed elevated expression and production of endothelin-1 (ET-1) compared with control mice and cells. Antagonizing ET-1 reversed the phenotype of Gsk3b-CKO fibroblasts and mice. Thus, GSK-3β appears to control the progression of wound healing and fibrosis by modulating ET-1 levels. -
1980 Seventeenth Annual Report
AGRA INDUSTRIES LIMITED CORPORATE HEAD OFFICE: 1101 CN TOWERS, SASKATOON, CANADA, S7K 1J5 PHONE (306) 653-5163 TELEX 074-2496 1980 SEVENTEENTH ANNUAL REPORT FINANCIAL HIGHLIGHTS Sales ............................................ Net Earnings - After Taxes Before Extraordinary Items ....... After Extraordinary Items .......... Net Earnings Per Share Before Extraordinary Items ....... After Extraordinary Items .......... Cash Flow ....................... ......... Cash Flow Per Share .................... Equity Per Share .......................... Average Shares Outstanding ........ Return on Equity ........................... CONTENTS Page Report to the Shareholden ............................ 4 Management Reports on Operations ............. 7 Ten Year Review ........................................... 14 Auditors' Report 16 Statement of Earnings ................................... 17 Balance Sheet Company Directory ANNUAL MEETING The annual meeting of shareholden will be held at 230 p.m. on Thursday, January 15, 1981 in the Sheraton West Room, Sheraton Cavalier Hotel, in Saskatoon, Saskatchewan. If you cannot be present, please vote by proxy. Beer Precast are erecting ceiling panels, roof panels and balustrades for the magnificent new Massey Hall in Toronto. AGRA INDUSTRIES LIMITED BOARD OF DIRECTORS D. H. C. BEACH Nipawin F. D. McCARTHY Edmonton G. H. BEATTY Regina T. A. McLELLAN Saskatoon J. S. BURTON Regina C. ROLES Saskatoon S. J. HAMER Vancouver H. TENENBAUM Toronto W. B. MANOLSON Toronto B. B.TORCHINSKY Toronto OFFICERS AND CORPORATE MANAGEMENT B. B. TORCHINSKY President and Chairman of the Board T. A. McLELLAN Executive Vice-President and Secretary A. W. BEAN Vice-President. Special Investments H. TENENBAUM Vice-President. Foods Group F. D. McCARTHY Vice-President, Engineering Group W. 8. MANOLSON Vice-President. Community Services Group K. J. TAYLOR Vice-President, Beverages Group W. V. FURBER Vice-President. Communications Division (Radio) R. G. DITTMER Treasurer 0. -
Windspeaker April 26, 1993
QUOTABLE QUOTE "What a lot of people want to do is keep us in a museum, saying this is what Native art must look like." - Paul Chaat Smith See Regional Page 6 April 26, 1993 Canada's National Aboriginal News Publication Volume I I No. 3 $1.00 plus G.S.T. where applicable Preserving traditions What better way to pass on culture than to celebrate it at a powwow? George Ceepeekous (right) and Josh Kakakaway joined people of all ages to dance at the Saskatchewan Federation Indian College powwow in Regina recently. People from all over Canada and the United States attended the powwow, which ',-ralds the beginning of the season. To receive Windspeaker in your mailbox ever two weeks, just send a reserve lands your cheque of money order in Act threat to the amount of$28 (G.S.T. By D.B. Smith over management of Indian re- would be able to find adequate community." land leg- Windspeaker Staff Writer serve lands to First Nations. funding for land development But similar charter Bands exercising their "inherent and management, said Robert islation in the United States led W1t authority" to manage lands un- Louie, Westbank First Nation to homelessness for many Na- 15001 the chief and chairman of the First tive groups because they mis- EDMON VANCOUVER der the act can opt out of land administration section of Nations' land Board. managed funds, Terry said. Natives across Canada are the Indian Act and adopt their "It would give them com- When the time came to repay outraged with the federal gov- own land charter. -
Halifax, Ns May 10-12 2018 Lord Nelson Hotel & Suites 1515 South Park Street
PROGRAM HALIFAX, NS MAY 10-12 2018 LORD NELSON HOTEL & SUITES 1515 SOUTH PARK STREET DURTY NELLY’S A Thursday, May 10 // 9:00pm // 1645 Argyle Street EAST OF GRAFTON INFORMATION B Friday, May 11 // 9:00pm // 1580 Argyle Street MAP A B CALM Conference information Lord Nelson Hotel and Suites 1515 South Park St. CALM Conference information Lord Nelson Hotel and Suites A: Durty Nelly’s Thursday, May 10 (9:00 pm) 1515 South Park St. 1645 Argyle St. A: Durty Nelly’s B: East of Grafton Thursday, May 10 (9:00 pm)Friday, May 11 (9:00 pm) 1645 Argyle St. 1580 Argyle St. B: East of Grafton 2 Friday, May 11 (9:00 pm) 1580 Argyle St. WHAT’S IN STORE 4 // Welcome to Halifax 6 // Resources 8 // Hotel Map 10 // Conference Schedule 11 - Overview 12 - Detailed Schedule 13 // Thursday, May 10 14 // Friday, May 11 22 // Saturday, May 12 30 // Award Judges 33 // AGM Agenda 3 WELCOME TO HALIFAX It’s been more than a decade since we last gathered here at a CALM conference. We’re excited to bring labour communicators together in this wonderful city, where the labour movement has been active and fighting for the rights of Haligonians and workers across the province. The 2018 CALM Conference’s exciting line-up will give every delegate the opportunity to sharpen old skills and learn new ones. While you can expect to participate in workshops that build on the basics, we know that CALM members are being asked to do more sophisticated communications work, often without additional resources. -
Dear City of London City Councillors, I Submit This Written Statement to You
Dear City of London City Councillors, I submit this written statement to you as I was unable to attend the Public Participation Meeting on Wednesday, March 20th, 2019 at Centennial Hall as part of the Special Strategic Priorities and Policy Committee Meeting regarding projects to be put forward for consideration for funding under the Government of Canada’s Infrastructure Canada Public Transit Infrastructure Stream (PTIS) funding program with a bilateral agreement with the Government of Ontario. Through the Public Transit Infrastructure Stream there is a shared goal between municipalities, the Government of Ontario and the Government of Canada that the Public Transit Infrastructure Stream will provide provinces, territories and municipalities with funding to address the new construction, expansion, and improvement and rehabilitation of public transit infrastructure, and active transportation projects. These investments will help to improve commutes, cut air pollution, strengthen communities and grow Canada’s economy. It is vital that the city of London have a strong and stable public transit system. The city of London is a city that is within the top 10 biggest cities in Canada by population size. We need a public transit system that is strong, stable and innovative to reflect our size and our needs. We are a mid to large size city that will only continue to grow with our prime location as a hub for Southwestern Ontario and a major artery to the Greater Toronto Area. We need to be forward thinking and bold in our approach to public transportation. Improving public transit encourages more people to take transit- improving the environment and our city and reduces commute and travel times for those who drive their own vehicles with a reduction of overall vehicles that are on the roadways. -
Hall of Fame
HALL OF FAME 2020 EDITION National Library of Canada Cataloguing in Publication Data Main entry under title: City of Orillia Hall of Fame 2020 edition Library and Archives Canada Cataloguing in Publication Isabel Brillinger, 1916-2011 author Commemorative Awards Committee / Kym Kennedy / Ellen Cohen -- 2020 edition. ISBN 978-0-9689198-2-8 (paperback) 1. Orillia (Ont.)--Biography. 2. Awards--Ontario--Orillia. I. Orillia (Ont.). Commemorative Awards Committee, issuing body II. Title. FC3099.O74Z48 2020 971.3’17 C2020-905298-X HALL OF FAME 2020 Edition Updated by: The Commemorative Awards Committee City of Orillia Cover Art by: Jieun Kim Introduction The Orillia Hall of Fame was established in 1964 to recognize residents, or past residents, of Orillia and area for their outstanding accomplishments. The award serves to build upon the history of our city and the incredible patrons who have built its past and present. Those nominated have received national and/or international recognition in their field of work or endeavour. Nominees have included those in the arts, professions, politics, business, philanthropy, athletics and more. In all cases, the nominees and, ultimately the inductees, have made a substantial impact on the destiny of Orillia. In order to ensure the legacy of the deeds and achievements of our Orillia citizenship, we invite nominations of those who inspire and illuminate. Details regarding criteria and deadlines are available at orillia.ca/halloffame. Take some time to visit the display of the 50+ inductees at the Orillia City Centre in the hall outside of the council chamber. 4 Chair’s Remarks Orillia isn’t just a beautiful city. -
Alan Earp: the President Reflects on 20 Years CONTENTS SURGITE Summer 1988
A newsmagazine for Brock alumni and the University community Summer 1988 Alan Earp: The President reflects on 20 years CONTENTS SURGITE Summer 1988 Brock Briefs -students get BIRT on the line so they don 't have to stand in one.... ...... 2 Ala n Earp The President retires Jul y 1, after 20 years at Brock........... ........... ..... .... 4 The Unisys Connection Brock's deal with the giant computer company.. ......... .. .. ...... ............ ..... 8 T he Alu mni Board Profil es of the members.. .. .... ..... .......... .. .... ... ..... ........................ ... .. .... ..... 13 Al umnews Wh o's doing what.. ... ............ .. ... .... .... ...... ..... .... .. ................. .. .. ............ .. .. 18 S urgite!/sur-gi-tay/v Latin I Push on! The last words of a dying hero and the motto of the thriving academi c institution that bears hi s name-Brock University, offers programs in th e arts, sci- ences, humanities, administrati ve studies, physical educati on, leisure studies and education. Brock University Chancellor Robert Welch Chairma n Board of Trustees Allan Orr Pres ident A lan Ea rp Presid ent Designate Terrence White Surgite is a qua rterly publication of the Office of External Relations. Director: Grant Dobson Editor: Janice Paskey The Cover photogra ph of President Ala n Earp was taken by Stephen Dominick, BAd min. '82. Stephen puts his degree to good use managing and marketing his own photography studio in St. Catharines. Corres pondence is welcome, write: Surgite, Office of External Relations, Brock Uni ve rsity, St. Catharines, L2S 3A l. The layout this issue was done by Heather Fox. 1 BIRT Not only is Brock the first university in Ontario to have Dial-A-Class hits Brock telephone registration, but we are the first in the world to use a synthetic voice, something like Arnold Schwarzenegger Brock Brie s according to one student. -
Pubuc Archives of Canada
PUBUC ARCHIVES OF CANADA MANUSCRIPT DMSlON • • PRELIMINARY INVENTORY RECORD GROUP 8 BRITISH MlUTARY AND NAVAL RECORDS 1954 r PUBLIC ARCHIVES OF CANADA • MANUSCRIPT DIVISION PRELIMINARY INVENTORY RECORD GROUP 8 BRITISH MILITARY AND NAVAL RECORDS 1954 This preliminary inventory co"ers all the British milil.l.f"y and naval records transferred to the Public i'rchives up to January, 1954. The measurements given indicate the linC'M space occupied on the shelves. CONTENTS RECORD GROUP 8 BRITISH MILITARY AND NAVAL RECORDS PAGE IXTRODLJCTIO~ . 5 J. C. SERIES (BRITISH :\1IL1T.-\RY RECORDS) A. Correspondence of the :\Iilitary Secretary of the Commander of the Forces. 1767-1870 7 B. Records of the Canadian Command, 1785-1883... 12 C. Records of the ;\ova Scotia Command, 1762-1899. 14 D. :\Iiscellaneous Records, 1757-1896 _.... 18 E. :\Iissing and Transferred \·olumes................ 20 11. OROX.\NCE RECORDS . 21 Ill. AD:\IIR.-\LTY RECORDS A. .'\dmiralty Lake Service Records, 1814-1833. 23 B. Admiralty P3cific Station Records, 1858-1903.... 25 INDEX . 27 3 97308-2 INTRODUCTION From 1759 until 1871 the defence of Canada was primarily the responsibility of British military and IIJ,val forces. However. in ISil Britain decided to recall most of her troops st'ltioned in Canada and left only a garrison at Ilalifax for the protection of the naval station. A similar garrison was later sent to Esquimalt. Early in the twentieth century increasing tension in Europe led Britain to concentrate a larger proportion of the Royal Navy in home waters. As a result the garrisons at Halifax and Esquimalt were recalled. -
Proquest Dissertations
EVALUATING THE EFFECTS OF WASTEWATER TREATMENT ON MARINE SEDIMENT CHEMISTRY IN HALIFAX HARBOUR, NOVA SCOTIA by Gwen A. Williams Submitted in partial fulfillment of the requirements for the degree of Master of Science at Dalhousie University Halifax Nova, Scotia July 2010 © Copyright by Gwen A. Williams, 2010 Library and Archives Bibliotheque et 1*1 Canada Archives Canada Published Heritage Direction du Branch Patrimoine de I'edition 395 Wellington Street 395, rue Wellington Ottawa ON K1A 0N4 Ottawa ON K1A 0N4 Canada Canada Your file Votre refdrence ISBN: 978-0-494-69842-6 Our file Notre reference ISBN: 978-0-494-69842-6 NOTICE: AVIS: The author has granted a non L'auteur a accorde une licence non exclusive exclusive license allowing Library and permettant a la Bibliotheque et Archives Archives Canada to reproduce, Canada de reproduire, publier, archiver, publish, archive, preserve, conserve, sauvegarder, conserver, transmettre au public communicate to the public by par telecommunication ou par I'lnternet, preter, telecommunication or on the Internet, distribuer et vendre des theses partout dans le loan, distribute and sell theses monde, a des fins commerciales ou autres, sur worldwide, for commercial or non support microforme, papier, electronique et/ou commercial purposes, in microform, autres formats. paper, electronic and/or any other formats. The author retains copyright L'auteur conserve la propriete du droit d'auteur ownership and moral rights in this et des droits moraux qui protege cette these. Ni thesis. Neither the thesis nor la these ni des extraits substantiels de celle-ci substantial extracts from it may be ne doivent etre imprimes ou autrement printed or otherwise reproduced reproduits sans son autorisation. -
Federation of Ontario Naturalists Radio
RADIO AS A VEHICLE FOR INFORMED ENVIRONMENTAL COMMENTARY - A FEASIBILITY STUDY A project proposal submitted by the Canadian Environmental Law Research Foundation and Federation of Ontario Naturalists April, 1984 CIELAP Shelf: Canadian Environmental Law Research Foundation; Federation of Ontario Naturalists Radio as a Vehicle for Informed Environmental Commentary - A Feasibility Study RN 27318 RADIO AS A VEHICLE FOR INFORMED ENVIRONMENTAL COMMENTARY A FEASIBILITY STUDY TABLE OF CONTENTS PAGE 1. The need for informed commentary 1 2. Why radio' 3 3. Study objectives 4 4. The study 5 5. Workplan 8 6. Timeline 9 7. Budget 10 8. The study team 11 9. The Canadian Environmental Law Research Foundation 35 10. The Federation of Ontario Naturalists 37 11. Appendices A) CJRT - FM Open College 48 B) The pilot programs 53 C) List of stations to be approached 57 D) Questionnaire 73 E) Letters of support 76 - 1 - THE NEED FOR INFORMED COMMENTARY The Canadian Environmental Law Research Foundation and Federation of Ontario Naturalists are pleased to submit this proposal for a study of the feasibility of producing a regular weekly radio program which would provide Ontario listeners with informed and relevant discussion of current environmental issues. Environmental problems continue to worsen - toxic chemicals increasingly threaten drinking water sources, acid rain is killing more and more northern lakes, while environmental contamination in the home and work place is a source of growing concern. Public reaction in the face of these mounting problems can be characterized by two words - concern and confusion. Public opinion polls show that Canadians believe strongly in the need for environmental protection. -
2019-2020 Report
CRITICAL RE-ACTION NAVIGATING CHALLENGING TIMES THROUGH LEADERSHIP AND PROFESSIONALISM 2019/2020 ANNUAL REPORT BIG CHALLENGES. TIMELY RESPONSE. 2019/2020 ANNUAL REPORT For the period September 1, 2019 to August 31, 2020 President’s Message ................................. 3 Registrar’s Message .................................. 4 About the College ...................................... 5 Highlights .................................................. 6 Registrant Statistics .................................. 8 Registration Committee ............................ 11 Quality Assurance Committee ................... 13 Complaints and Discipline ......................... 15 Patient Relations ..................................... 17 College Council and Staff ......................... 18 Financial Statements ............................... 20 2 CKO 2019-2020 ANNUAL REPORT PRESIDENT’S MESSAGE RESPONDING TO CHANGING EXPECTATIONS Council is the College’s board of directors. It sets the strategic and policy direction, and ensures that the College is acting in the public interest. Part of this important duty means that we monitor and respond to issues impacting the patients/clients of kinesiologists and kinesiologists themselves. In the spring of 2020 when communities near and far marched in a global In the coming months we will develop and publish a profile of qualifications demand for justice and equality, the College acknowledged this important that all potential Council members must meet if they wish to serve on Council. fight. As an organization